Reviews of Geophysics, 34, 1-31, 1996
David P. Stern, Laboratory for Extraterrestrial Physics
Goddard Space Flight Center, Greenbelt, MD 20771
Abstract
After 1958, when scientific satellites began exploring the Earth magnetic environment, many puzzling phenomena could be directly examined, especially the polar aurora and disturbances of the Earth's magnetic field [see Stern, 1989a]. The notion of the solar wind, introduced that same year, helped clarify the role of the Sun in driving such phenomena. The large-scale structure of the magnetosphere, the space region dominated by the Earth's magnetic field, was gradually revealed within the next decade: its trapped particles, its boundary, and its long magnetic tail on the night side. Inevitably, however, the new discoveries led to new questions at a more fundamental level, about the transfer of energy, the flow patterns of plasmas and electric currents, the acceleration of the aurora, and about transient events such as magnetic substorms and storms, which energized ions and electrons. Though significant progress has occured in some of these areas, many unresolved issues still remain. This review outlines the history of magnetospheric research, draws some general conclusions and provides an extensive bibliography.
Table of Contents
Clicking on any marked section on the list below brings up a
file containing it and all unmarked sections immediately
following it on the list. This list is repeated at the beginning of each file.
- Introduction
- Discovery of the Radiation Belts
- Artificial Belts and Early Studies
- The Large Scale Structure
- Convection
- Reconnection
- The Open Magnetosphere
- Observational Tests
- The Polar Aurora
- Field Aligned Voltage Drops
- Birkeland Currents
- Substorms: Early Observations
- Substorms: The Satellite Era
- Substorms: Theory
- Convection in the Geotail
- Planetary Magnetospheres
- Other Areas
- Assessment
References: A-G
References: H-P
References: Q-Z
Back to "Exploration"
1. Introduction
This is the second part of a concise history of observations of the Earth's magnetosphere and of their interpretation. It and the first part [Stern, 1989a, henceforth denoted BH-1] are meant to help trace the development of magnetospheric physics in a unified context and to outline its framework of observations and ideas. The early years, around 1957-64, are covered chronologically, after which the coverage is arranged by topics--convection, reconnection, aurora, Birkeland currents and substorms. At the end some overall trends are assessed, as well as the current state of the discipline. Readers who find this article too technical are referred to an exposition on the world-wide web by Stern and Peredo [1995].
This brief account is based primarily on work published in English, which covers US efforts fairly completely but is unfortunately far less detailed on space research in the USSR and elsewhere. The first part (BH-1) covered earthbound studies of the magnetosphere before artificial satellites were available, and here the rest of the story is presented. The concluding section contains an assessment of overall trends as well as of the current state of the discipline.
This account is mainly based on published source, rather than on personal papers or interviews. It must therefore be viewed as a mere framework, in which many details remain to be filled.
2. Discovery of the Radiation Belts
Early US magnetospheric research was mainly focused on the Earth's radiation belts, discovered by Van Allen and his colleagues in the spring of 1958. It drew from four sources. The first source was laboratory plasma physics, aimed at achieving nuclear fusion. Its early focus was in Princeton, where a number of "stellarator" confinement machines were built [Bishop, 1958], and it is interesting to note that James Van Allen, too, worked at the Princeton Plasma Lab in 1953-4 [Van Allen, 1983a, 1990]. Early plasma research provided an understanding of particle confinement in magnetic fields and of adiabatic invariants [Spitzer, 1956; Rosenbluth and Longmire, 1957; Northrop and Teller, 1960], essential to the theory of the radiation belts.
The second source was high altitude research on radiation in space, mainly cosmic rays, using balloons and rockets [Friedman, 1994]. Balloon studies of the primary cosmic radiation started shortly after World War II [Simpson, 1994]; they led among other things to the discovery of the pion (pi-meson), and they were greatly expanded towards the IGY, the International Geophysical Year [Van Allen, 1983b; Odishaw and Ruttenberg, 1958]. Rocket-borne studies began shortly after the end of WW-II, when captured German V-2 rockets were brought to the US and were used there for high-altitude research [DeVorkin, 1992], and they continued with vehicles specifically designed for science, in particular the "Aerobee" [Newell, 1959]. Rocket instruments of the University of Iowa were launched in 1954 towards the aurora and their particle counters registered the presence of radiation [Meredith et al., 1955], later credited to x-rays produced by the electrons in the rocket shell or the atmosphere [e.g. Van Allen, 1995]. On the first day of the IGY balloon-borne instruments of the University of Minnesota also observed X-rays produced by auroral electrons, which penetrated deeper into the atmosphere than the electrons themselves [Winckler et al., 1957, 1958].
The third source was interest in high-energy particles originating at the sun. This interest was given a great boost by the large solar particle event of February 23, 1956, which registered on cosmic ray detectors around the world. Solar particle research was also one of the active foci of the IGY (7.1.57-12.13.58), which coincided with a peak in the sunspot cycle. It was believed at the time that such particles were energized in solar flares by processes involving magnetic fields [Giovanelli, 1947; Hones, 1984b], and this stimulated the developments of theories of particle acceleration. Many of the results developed in this context, especially those of "reconnection theory" (see below), were later applied to the magnetosphere.
A fourth source was the study from the ground of the aurora and of magnetic variations. Its community included Sydney Chapman, James Heppner, Ernest Vestine and Neil Davis, and was initially rather loosely coupled with the space observervations. Soon, however, it contributed in major ways to their interpretation.
Sputnik 1 was launched October 4, 1957, followed on November 3 by Sputnik 2; Explorer 1, the first successful US spacecraft, was launched January 31, 1958, and Explorer 3, by which the radiation belt was discovered, was orbited March 26.
Sputnik 1 carried no radiation detector, but Sputnik 2 did so, rising to an altitude of 1680 km. S.N. Vernov actually reported a significant (though not overwhelming) increase of the radiation rate between 500 and 700 km and in hindsight, this apparently marked the fringes of the radiation belt; however, he did not realize the implications [Singer, 1962; p. 249-258]. The apogee of Sputnik 2 was above Australia; the Australians who tracked it there asked the USSR for the key to its signals but were refused, and hence the data were not analyzed at that time [Hess, 1968, p.11; Dessler, 1984].
The mission of Explorer 1 was assembled in a hurry. The US was trailing the USSR in space exploration, and the first attempted launch of its official entry, the Vanguard, ended in flames on the launching pad, and a launch vehicle was therefore improvised from available components, a back-up plan devised earlier by Von Braun [1964; Pickering, 1963]. The resulting orbit was rather non-circular and rose to an apogee of 2500 km, deep inside the radiation belt. Explorer 1 carried a radiation detector, a Geiger counter provided by Van Allen's team at the University of Iowa (Figure 1), which also included Carl McIlwain, Ernie Ray and George Ludwig [Van Allen, 1981, 1983a]. The counter was meant to measure the overall cosmic ray intensity, which by Stoermer's theory [see BH-1] was expected to increase with magnetic latitude, and its predicted counting rate was about 30 counts per second. The experimental package was a modified version of one designed for a later launch in the Vanguard series, and included a tape recorder designed to store the data for retransmission when the spacecraft passed over tracking stations. However, it was decided to provide this feature only on later missions and to omit it from Explorer 1, so that stations were only able to collect a few minutes' worth of data whenever Explorer 1 passed within range.
| 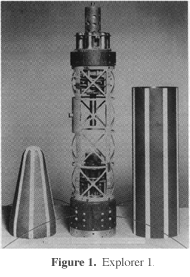
|
On passes below 600 km the counting rate was nominal. Near apogee, however, no counts at all were detected, and on one pass, with the spacecraft around 1200 km and rising, counts were received but suddenly stopped [Van Allen, 1981, Fig.8]. Actually, much of this was only noted later: what was mainly noted was that sometimes the counter on the spacecraft operated normally and at other times it seemed dead. McIlwain showed experimentally that very high particle fluxes would overwhelm the counter and produce zero counts, and there is little doubt that further analysis of Explorer 1 data would probably have led to the discovery of the radiation belt. Before that could happen, however, much less ambiguous data were obtained from another experiment.
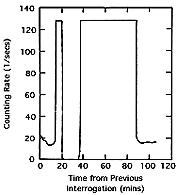
Figure 2. Counting rate of Explorer 3 during a pass through the radiation belt. The highest rate was encoun- tered during the segment of zero counts.
| Explorer 2 failed to orbit, but Explorer 3 was successful. Unlike Explorer 1, it carried a tape recorder, and its continuous record of data (Figure 2) made clear what was happening. At low altitudes, only cosmic rays were detected; then as the satellite rose the recorded counting rate increased up to the highest it could record, and it stayed pegged there for a while. At a still higher altitude it abruptly fell to zero, and during descent the same transitions occurred in reverse order. The periods of zero counts near apogee clearly marked not the absence of radiation but a very high radiation flux: the Geiger counter was discharged so frequently that it did not recover between pulses and its output signals decreased until they no longer triggered the counting circuit.
|
That was how the radiation belt was discovered [Van Allen et al., 1958; Van Allen, 1981]. Ernie Ray's comment was: "My God, space is radioactive!" [Hess, 1968]. The identity of the particles was quite uncertain. Auroral electrons had been observed in near-Earth space, but they lacked the energy to penetrate the counter walls. They could trigger a count by means of secondary X-rays, with a probability of the order of 10-5 [Frank, 1962], an explanation which had been previously used to explain rocket observations in the auroral zone [Van Allen, 1995]. Such an interpretation would have implied a huge flux of electrons, of order 100 million per cm-sq. sec, a figure which proponents of manned space flight viewed with justified alarm. Sputnik 3, launched May 12 to an apogee of 1880 km, carried scintillation detectors and confirmed the existence of Van Allen's belt, but it did not resolve the identity of the particles, and neither did Explorer 4, discussed in the next section [Van Allen et al., 1959]. As noted further below, the particles of the intense innermost part of the belt were soon accounted for. It was later pointed out by Dessler [1960; also Dessler and Vestine, 1960] that the extremely high fluxes, implied by the X-ray interpretation, would have modified the Earth's field above and beyond the changes actually observed. However, the uncertainty about the outer belt persisted until the work of Davis and Williamson [1963, 1966] and Frank's electron measurements with OGO 3 [Frank, 1967].
In conclusion, it should be stressed here that the above is a very abbreviated summary of a complex period of discovery, and a much more detailed picture is available from Van Allen [1983a].
3. Artificial Belts and Early Studies
Explorer 4 was built in a record 77 days from initiation to its launch on July 26, 1958, and it had two goals: to study the new radiation belt in greater detail, and to observe an artificial radiation belt produced by high-altitude nuclear bombs, a project of the US Air Force code-named Argus. The idea originated with Nicholas C. Christofilos, a Greek engineer who on his own had studied the motion of charged particles in magnetic fields [Foster et al., 1973]. Before the principle of strong focusing was incorporated in high-energy accelerators, Christofilos proposed it independently but was ignored. He came to the US in 1953, started working at the Lawrence Livermore Lab in 1956 and there he proposed the Argus experiment, designed among other things to test on a large scale the confinement of charged particles in magnetic fields.
Three small bombs, carried by rockets launched from US Navy ships [Christofilos, 1960] were detonated 100-500 km above the South Atlantic on 8.27, 8.30 and 9.6.58. Because of the remoteness of the site, the project remained a secret until its results were released the following year. Scientifically it was a great success. Artificial aurora was observed at the other end of the field line (near the Azores) and Explorer 4 recorded artificial belts of high-energy electrons which decayed within a few weeks [Van Allen and Frank, 1959; Hess, 1968].
Up to this time all explanations of the radiation belt linked it to the aurora, but in the second half of 1958 at least three investigators independently conceived a different explanation, related to cosmic rays: Singer [1958], Kellogg [1959] and Vernov [1959]. Cosmic rays are rapidly moving atomic nuclei (mostly protons) whose energies start in the GeV range and extend in diminishing numbers much higher. They arrive at the solar system from distant space and when they colide with nuclei of the atmosphere they produce a spray of secondary fragments.
Most secondary particles from such collisions move earthward and are lost, but a small fraction ("albedo") is splashed away from the Earth. If an electrically charged albedo particle has high energy it either escapes or else is guided by magnetic field lines to the opposite hemisphere, where it is usually absorbed by the atmosphere. An albedo neutron however moves unimpeded until it decays into an electron, a neutrino and a proton, with the latter receiving almost all the kinetic energy.
The mean decay time of a free neutron is about 10 minutes, but actual times for individual neutrons are distributed statistically and for a typical fragment energy of 20-50 MeV, a few neutrons may already decay within the first few hundredths of a second. The decay protons may then materialize deep enough in the magnetic field to remain trapped there, and their lifetime in such trapped orbits is long enough to allow an appreciable density of energetic protons to accumulate. This was what Singer, Kellogg and Vernov proposed, and the existence of such protons was confirmed in 1959 by nuclear emulsions flown aboard rockets into the near-earth radiation belt and later recovered [Freden and White, 1959; Hess, 1962; White, 1966, 1973].
In hindsight, it was realized much later that powerful shocks, created inside the magnetosphere by interplanetary shocks of solar origin, could on rare occasions accelerate protons near Earth to energies of many MeV. Thus the event of March 24, 1991 was observed to create a belt of 20 MeV protons just outside the inner belt, of comparative intensity [Blake et al., 1992]. It is possible that a similar event produced the double-peaked structure of the inner belt observed by Explorer 15 [McIlwain, 1963].
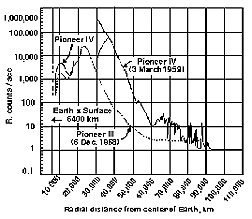
| Trapped radiation was however observed at much greater distances than albedo theory could explain. After the December 1958 flight of Pioneer 3, Van Allen and Frank [1959] concluded that there existed not one belt but two (Figure 3)--an "inner belt" created by albedo neutrons and an "outer belt" then believed to consist of energetic electrons from some other source, extending to distances of 6-8 RE (Earth radii) [Farley, 1963; O'Brien, 1963]. The outer belt was studied by Explorer 6, launched 7 August, 1959 [Naugle, 1965].
|
Figure 3. Counting rates of Pioneers 3 and 4, during traversals of the outer radiation belt [Van Allen and Frank, 1959].
Yet another particle population was added on July 9, 1962, when the Defense Atomic Support Agency (DASA) and the Atomic Energy Commission (AEC) exploded a hydrogen bomb (a project code-named Starfish) in the inner belt region. The bomb created an intense belt of MeV electrons [Brown et al., 1963] which among other things damaged the solar arrays of satellites and caused three of them to fail soon afterwards. Some Starfish electrons persisted for five years [Hess, 1968], underscoring the long lifetime of particles in the inner belt. NASA was caught by surprise: it retrieved an engineering model of Explorer 12 from one of its museum displays, hurriedly turned it into a regular satellite and launched it as Explorer 15, all within 91 days [Corliss, 1967, p. 728]. The Department of Defense reacted even more quickly, launching Starad (1962bk; see Corliss [1967], p. 790) within 60 days of the decision to launch the mission (M. Walt, personal communication, 1995). Before the international test ban took place, Soviet Russia also exploded three large bombs, but these tests occurred on more distended field lines and their radiation belts only lasted a number of weeks [White, 1966].
At first it was held that the aurora was simply the overflow of the outer belt:
"... we propose that the radiation belt is the reservoir whose leakage of particles is the direct cause of visible aurora. It is further suggested that solar plasma replenishes the reservoir from time to time, working its way into the outer reaches of the earth's magnetic field when its density is sufficiently great, then being trapped in the field." [Van Allen et al., 1959].
This was known as the "leaky bucket" theory, the bucket being the belt and the leak the aurora [O'Brien, 1967; Kennel, 1969, Fig. 15]. Later it was pointed out [Dessler, 1960] that the high electron fluxes attributed to the outer belt should have significantly deformed the magnetic field. An experiment aboard Explorer 12, designed to resolve the problem, showed a large flux of positive ions, presumably protons [Davis and Williamson, 1963, 1966], energetic enough to penetrate the counters; this component later turned out to contain most of the energy. The outer belt also contained energetic electrons, but their intensity fell far short of the level needed to produce the aurora [O'Brien, 1962, 1964]. Opinion then shifted to the alternative "splash catcher theory" by which some outside mechanism was producing auroral electrons and the outer belt was merely a by-product, incidental splash captured from the main torrent.
4. The Large-Scale Structure
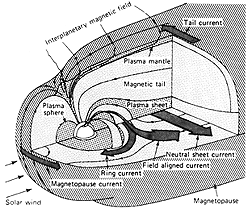
| The picture became much clearer during 1962-5 [LeGalley, 1963], when the large-scale structure of the Earth's magnetic environment (named "magnetosphere" by Gold[1959]) was established (Figure 4). This phase followed the observational confirmation of the existence of the solar wind, predicted by Parker (see BH-1). In 1962 Explorer 12, on the sunward side of the Earth, repeatedly crossed a sharp boundary between the magnetosphere and the solar wind, named the "magnetopause" [Cahill and Amazeen, 1963; Cahill, 1995; Hines, 1963; Hess et al., 1965; Dungey, 1978] and resembling in many ways the boundary predicted (for storm times) by Chapman and Ferraro (see BH-1).
|
Figure 4. Regions and currents of the Earth's magnetosphere, representing the state of our knowledge around 1970 [Lopex and Baker,1994]. A small normal field component on the magnetopause allows some interplanetary field lines to link up with the Earth's field.
The following year Explorer 18 (or IMP 1, for Interplanetary Monitoring Platform) observed a collision-free bow shock ahead of the magnetopause [Ness et al., 1964], predicted by Axford [1962] and Kellogg [1962], and also established the properties of the long geomagnetic tail [Ness, 1965, 1987], briefly glimpsed in 1961 during the 52-hour mission of Explorer 10 [Heppner et al., 1963]. Those observations and others by Explorer 14 and the Vela satellites led to two predictions of a "tail" of the magnetosphere (Ness [1969], p. 100). Dessler and Juday [1965] predicted two bundles of magnetic flux trailing behind the Earth's poles, twisted by the Earth's rotation, with a current profile resembling the Greek letter theta. Axford et al. [1965] predicted similar "tail lobes" but also suggested a layer of plasma in the equatorial region between them, carrying a cross-tail current which then closed around the tail magnetopause, also with a q-profile. They furthermore suggested that the distant neutral line predicted by Dungey ([1991]; sect. 7, below) was embedded in that plasma sheet.
IMP 1 and later IMPs confirmed Axford's plasma sheet, finding it typically
2-8 RE thick, with a dawn-to-dusk electric current of order 200,000 A/Earth Radius , and a plasma of about 0.5 ions/cc of ions of 1-5 keV and electrons around 1 keV. The plasma of the tail lobes was very rarefied, typically 0.01 ion/cc.
The tail contained two low-density "tail lobes", bundles of roughly parallel field lines directed sunward in the northern lobe and anti-sunward in the southern lobe, as required for field lines connected to the appropriate polar caps of the Earth (compare Dessler and Juday [1965]). Sandwiched between the lobes was a near-equatorial "plasma sheet." The fact that the sheet was bounded by oppositely directed magnetic fields showed that it carried a dawn-to-dusk electric current of the order of 200,000 A/RE, and this current closed around the magnetopause, giving the entire flow pattern the profile of the Greek letter theta [Axford et al., 1965; Axford, 1994]. I. Axford (personal communication, 1992) has claimed that this work predated the disclosure of IMP 1 results.
The earthward edge of the plasma sheet contained ions of about 20 keV, and these blended continuously with the ions of the ring current [Frank, 1971]; on the other hand, the energetic electron population of the sheet [Vasyliunas, 1968] displayed an asymmetrical inner edge. (Because of the presence of low-energy electrons, this does not contradict the plasma's electrical neutrality.) The inner edge was originally credited to electrons precipitated into the atmosphere by plasma instabilities [Kennel and Petschek, 1966] but in hindsight it might also be related to region 2 Birkeland currents (below), carried almost entirely by electrons.
The existence of a "ring current" circling the earth during magnetic storms was inferred long before the spaceflight era, from the magnetic signatures of such storms, and was the subject of extensive speculation [Smith, 1963]. Observations in space indicated that it was carried by trapped particles of relatively low energy, as had been suggested by Singer [1957], and that it was not a transient effect of storms but a permanent feature. It was studied (launch dates given as (mo.day.yr)) by the Orbiting Geophysical Observatories [Ludwig, 1963; Jackson and Vette, 1975] OGO-1 (9.5.64), OGO 3 (6.7.66) and OGO 5 (3.4.68), later by Explorer 45 ("Small Scientific Satellite" or S3; (11.15.71)) [Longanecker and Hoffman, 1973] and by the European GEOS 1 (4.20.77) and GEOS 2 (7.14.78). Its energy is mainly carried by ions with energies around 50 keV [Frank, 1967], but its exact composition and energy (median value about 85 keV) were only mapped by the Charge Composition Explorer (CCE) of the AMPTE mission, launched in 1984 [Williams, 1987; Lui and Hamilton, 1992].
The ring current grows stronger during magnetic storms, and it was shown theoretically that the intensity of the magnetic disturbance observed at Earth is then very nearly proportional to its total energy [Dessler and Parker, 1959; Sckopke, 1966; Carovillano and Siscoe, 1973]. Between such injections the ring current slowly decays, mostly by charge exchange collisions [Liemohn, 1961; Smith et al., 1976] with atoms of the hydrogen cloud ("geocorona") surrounding the Earth [Hunten and Donahue, 1976 ; Carruthers et al., 1976]. Each collision produces a slow proton and a fast neutral atom; such high-energy atoms are able to trigger particle counters, and since they are (like albedo neutrons) unaffected by the Earth's magnetic field, it has been proposed to use them for remote sensing of the ring current [Williams et al., 1992]. In 1971 it was also observed that a sizable portion (about 15%) of the particles added to the ring current during a magnetic storm were O+ ions of atmospheric origin, evidence of a near-earth acceleration process [Shelley et al., 1972].
A special region is the polar cusp, the region inside the high-latitude dayside magnetopause which separates field lines that close near the "nose" of the magnetosphere from those swept into the tail. The cusp was first explored in 1969-72 by the European spacecraft HEOS 1 and HEOS 2 (and later by Iowa's Hawkeye-1) which found a region of disordered weak magnetic fields [Mencke-Hansen, 1976]. Such fields are unable to exclude the solar wind, or more accurately, the magnetosheath plasma, a name given by Dessler and Fejer [1963; see Fig 1 there] to the solar wind plasma slowed and heated by passage through the Earth's bow shock. It is therefore filled with magnetosheath plasma, which spills into a funnel-shaped region reaching all the way to the atmosphere and produces a characteristic auroral glow [Shepherd, 1979].
The HEOS satellites also discovered a variety of boundary layers just inside the magnetopause, with a thickness of the order 0.3-1 RE, typical density 1-3 cm-3 and typical tailward velocity of 100-150 km/sec. A narrow "low latitude boundary layer" was found on the day side [Paschmann et al., 1976] and may be the result of reconnection processes (below) near the nose of the magnetosphere, though other origins are also possible. The much thicker "plasma mantle" found at high latitudes tailward of the cusp [Rosenbauer et al., 1975] may be formed by sheath plasma crossing an Alfvenic transition extending from the site of magnetic reconnection, discussed further below [Levy et al., 1964] , and it gradually widens with increasing distance from Earth [Hardy et al., 1975, 1979].
In what follows, coordinates in the magnetosphere will be given in the so-called geocentric solar magnetospheric coordinate system (GSM), described by Russell [1971; also Hapgood, 1992]. In these coordinates, the x-direction points to the Sun, the x-z plane contains the Earth's dipole axis and north is on the positive side of the plane z=0. The x-direction is also assumed to be the one from which the solar wind is blowing, although in very accurate work the small aberration angle (about 4 degrees) due to the Earth's orbital motion is also taken into account. The actually observed direction of the solar wind may differ from this by a few degrees (in both elevation and azimuth) due to effects occurring closer to the Sun.
5. Convection
The processes producing the complex features of the magnetosphere must meet two requirements: sufficient energy must be available, and particles must somehow be accelerated to observed energies. As for the supply of energy, it was estimated [Axford, 1964; Stern, 1984] that about 1-2% of the solar wind energy impinging on the magnetopause cross-section is tapped by internal processes of the magnetosphere.
In the neutral atmosphere of the Earth, energy is usually transmitted by two mechanisms: by large-scale circulating flows which convect heat from the ground upwards, and by radiation which takes a more direct path. The magnetosphere, too, may transmit energy both by convective flows and by a more direct route, involving field-aligned currents.
In an ideal magnetized plasma, a steady bulk flow with velocity v requires the existence of an electric field E, satisfying the "ideal magnetohydrodynamic (MHD) condition" [e.g. Walen, 1946; Alfven, 1950]
E = - v x B (1)
Conversely, an electric field E impressed on a magnetospheric plasma produces a bulk flow satisfying (1). It is a property of (1) that "particles move with field lines", i.e. any group of ions or electrons sharing a field line at one time continues doing so ever after, and a "moving field line" in what follows will mean a moving string of plasma particles, threaded by a common field line. If dB/dt = 0, the magnetic configuration is fixed and on any "moving" line, the plasma population along its entire length migrates to an adjoining line: thus field lines can (for instance) transmit bulk motions from distant regions to their ionospheric ends. In inductive electric fields with dB/dt not zero, field line sharing also holds [Newcomb, 1958; Stern, 1966] but bulk motion is not necessarily transmitted along field lines [Stern, 1990, Figure 7].
The existence of the Chapman-Ferraro cavity (see BH-1) and hence of the magnetopause may be viewed as another consequence of field line sharing: as long as such sharing is rigorously enforced, there exists no way for interplanetary plasma, threaded (presumably) by fields of solar origin, to mix with plasmas of the Earth's field. For related reasons, as long as all terrestrial field lines are confined to the cavity's interior ("closed magnetosphere"), it is also difficult for energy, momentum and electric currents to enter the cavity from the outside. In the early days many scientists in fact believed that magnetospheric field lines were in this way completely confined inside the cavity. The alternative view of an "open" magnetosphere developed gradually and is discussed in sections 7 and 8.
Gold [1959, p. 1220] noted that the large scale flow of magnetospheric plasma (a type of which he was studying) "is quite analogous to thermal convection" and that led to the term "convection" used by Axford and Hines [1961] to describe large-scale circulation inside the magnetosphere, caused by the solar wind. The theory of Alfven [1939] (see BH-1, also Cowling [1942] and Stern, [1977]), although not consistently formulated, may also be viewed as a theory of magnetospheric convection. Contemporary theories began with Axford and Hines [1961, also Hines, 1974, p. 3, 933; Axford, 1962, 1964, 1994; Hines, 1964, 1986] and with the work of Dungey [1961] described further below. Axford and Hines proposed a convective circulation to explain an observed pattern of auroral motions [Davis, 1962, 1971] in which plasma seemed to circulate in the polar cap.
Axford and Hines visualized a magnetosphere whose field filled a cavity in the solar wind, elongated on its night side into a tail, as previously suggested by Johnson [1960], so that all field lines emanating near the magnetic pole extended into the tail. Their proposed convective flow pattern (Figure 5a, from Hill [1983])) carried plasma tailward along the flanks and returned it by means of a sunward flow near the x-axis, skirting around the region closest to Earth. They furthermore suggested that such a flow could be caused by a viscous-like momentum transfer from the solar wind to adjacent regions of the tail, although they admitted that other processes could produce similar flows in the polar cap, including Dungey's reconnection scenario (further below).
| 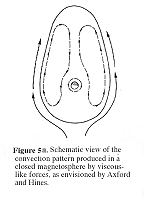
|
When the flow pattern of Figure 5a is mapped along field lines to the polar ionosphere, it produces a two-cell flow pattern, with plasma streaming nightwards across the pole and returning to the day side at lower latitudes, with flow lines similar to the contours in Figure 5b. By equation (1), if E =-grad V, it follows that v/gradV = 0 and therefore the plasma flow lines in Figure 5b are also lines of constant electric potential F, suggesting a dawn-to-dusk electric field across the polar caps. Satellites in a low-altitude polar orbit can observe such a field directly, by measuring the small voltage difference between the tips of a long antenna [Aggson, 1968; Cauffman and Gurnett, 1972]. The first satellites to successfully conduct such observations were Iowa's Injun 5 [Cauffman and Gurnett, 1971] and the OGO-6 observatory [Heppner, 1972a, 1977]; some later missions, e.g. Atmosphere Explorer 1 and Dynamics Explorer 2, measured E indirectly using "driftmeters" which observed v through the anisotropy of particle fluxes caused by the plasma's bulk motion [Hanson and Heelis, 1975; Heelis et al., 1981].
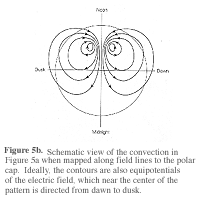
| The observations confirmed the two-cell pattern and obtained typical voltage drops of 40-70 kV; this agreed with a prediction of the reconnection model by Levy et al. [1964; sect. VI]. Much depends upon the state of the interplanetary magnetic field (IMF). The two-cell pattern is most stable when the IMF has a southward slant (Bz < 0, see below), and it contains asymmetries correlated with the dawn-dusk By component of the IMF [Heppner, 1972c; Heppner and Maynard, 1987]. The cross-polar voltage drop DF on the average grows with southward Bz, though individual observations fluctuate greatly. With northward Bz the average DF sometimes decreases to less than 20 kV, and it has been suggested that at such times it may "bottom out" at a low level contributed by a viscous-like interaction at the flanks [Reiff et al., 1981; Wygant et al., 1983].
|
When the IMF has a northward slant, the 2-cell pattern becomes distorted and E is often irregular. At times non-standard patterns may develop, such as the 4-cell pattern deduced by Burke [1979]. More complex patterns have also been claimed [Reiff and Burch, 1985] but they are hard to confirm without simultaneous passes by a fairly large number of satellites.
In the innermost magnetosphere the plasma density n is dominated by the thermal ionospheric plasma which tends to co-rotate with Earth; this is another consequence of field line sharing [Ferraro, 1937] and is enforced by a corotation electric field ECR, which near Earth is much larger than the convection field E. It was found from whistler wave observations [Carpenter, 1963; Carpenter and Park, 1973] and later by in-situ observations that n often dropped precipitously from about 20-100 ions/cc to about 5 ions/cc at a "plasmapause" boundary on field lines that extended to 4-5 RE. Brice [1967; Kennel, 1985] and Nishida [1966] proposed that this was essentially the boundary of the region where low energy plasma shared the rotation of the Earth; beyond it the convection electric field E overpowered ECR. This view is now widely accepted, although the suggestion was also made [Lemaire, 1975] that the plasmapause was the limit beyond which low-energy plasma was easily lost through the interchange instability, an approach first explored by Brice [1973].
6. Reconnection
As early as 1942 cosmic ray detectors observed the arrival of high-energy ions associated with solar activity, reaching at times up to approximately 10 GeV [Forbush, 1946; Pomerantz, 1984; Van Allen, 1993]. For many years such events were credited to solar flares, although recent evidence points to a much better correlation with coronal mass ejections [Gosling, 1993]; their most plausible energy source, then as now, seems to be the intense magnetic field of sunspots. It was speculated that somehow part of that field was "annihilated" by a rapid process and its energy used to accelerate ions and electrons, the latter revealed indirectly by intense bursts of radio noise, and more recently, by X-rays.
The process most favored for such energy release was magnetic merging or magnetic reconnection (synonymous terms). It may be loosely defined as a flow of plasma in which some of the field lines threading the plasma pass through a neutral point or neutral line at which the magnetic field vanishes. The idea originated with Giovanelli [1947; Hones, 1984b] and was then developed by Sweet [1958] and especially by Dungey [1953, 1963, 1994, 1995; Stern, 1986].
Figure 6
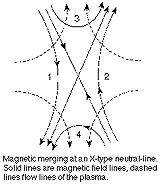
| The starting point is the observation that the field-line sharing property associated with equation (1) can be violated if plasma flows through a neutral point (Figure 6) where B=0 and where the field's direction is undetermined. In the X-type neutral point drawn here (actually a neutral line if this configuration extends unchanged into the third dimension) field lines cross in the pattern of the letter X and plasma arriving on field lines of the regions "1" and "2" depart on differently connected lines in "3" and "4". Dungey, Sweet and others proposed that this process might somehow modify the large-scale magnetic configuration and thereby release magnetic energy. Particles would be accelerated by the electric field associated with the motion, producing fast jets of plasma flowing away from the neutral line as the plasma exits on lines "3" and "4", and shocks which heat the plasma [Levy et al., 1964].
|
Additional effects must be invoked, for by (1), if E is finite and B tends to 0, the velocity v which particles need to keep up their field-line sharing property becomes infinite. Sweet [1950] showed that in conducting fluids with finite resistivity the plasma's motion lags behind that of field lines, and therefore reconnection theories have often assumed a finite (but small) resistivity in the region near B=0. Other processes which preclude an infinite v may also play a role, e.g. ion or electron inertia [Vasyliunas, 1975, table 2].
A somewhat different type of process ("group 2 merging" of Vasyliunas, [1975], p. 307) is illustrated by the collision of two bodies of plasma permeated by magnetic fields of equal intensity but opposing directions, separated by a "neutral sheet" of zero field intensity. The plasma may emerges as a narrow stream along the sheet, perpendicular to field lines, its magnetic field "annihilated" and its particles accelerated by the attendant E, though its electric neutrality may pose problems [Stern, 1990].
Magnetic reconnection is relevant to magnetospheric physics in two distinct ways: it makes possible a realignment of field line connections, e.g. the establishment of a linkage between the Earth's field and the IMF, and it may also release magnetic energy and accelerate particles. The first aspect is important to the concept of the open magnetosphere, the second to substorms, two items discussed separately further below.
As noted earlier, many researchers arrived at magnetospheric physics from the study of solar energetic particles, and they brought with them an interest in reconnection [Parker, 1963]. That led to a 1963 symposium at Goddard Space Flight Center [Hess, 1964] where, among other things, the theory of Petschek [1964, 1995] was presented, giving a more detailed scenario of the reconnection process. Important references to later work may be found in a comprehensive review by Vasyliunas [1975], in the proceedings of a 1984 conference at Los Alamos [Hones, 1984a] and in reviews by Sonnerup [1979] and by Forbes and Priest [1987]. Avenues explored nowadays include the relation to tearing instabilities in plasmas [Schindler and Birn, 1978], reconnection at multiple points [Lee and Fu, 1985] and relations to chaotic field line topology [Hesse and Schindler, 1988]. A growing number of studies simulate reconnection by means of fast computers.
There exists some evidence for reconnection from direct observations at the dayside magnetopause [e.g., Paschmann et al., 1979; Sonnerup et al., 1981] but it is difficult to verify details of the mechanism. In regions where reconnection seems likely to occur, magnetic fields are quite variable and with isolated spacecraft it is almost impossible to extract their structure. The existence of a rarefied "depletion layer" outside the magnetopause, seen only when the directions of B inside and outside are similar, is taken as evidence for reconnection. Claims have also been made that characteristic oscillations of the magnetic field observed near the dayside magnetopause, associated with southward IMF and termed "flux transfer events" [Russell and Elphic, 1978, 1979; Elphic, 1994], are local signatures of "patchy reconnection", but in spite of extensive studies, such events remain poorly understood.
7. The Open Magnetosphere
Reconnection was first applied to magnetospheric physics by Dungey [1961], as the key ingredient of his alternative theory of convection. Dungey proposed that an X-type neutral point (or line) at the front of the magnetosphere enabled terrestrial field lines to link up with interplanetary ones and produce "open" field lines, with one end on earth and the other in distant space.
| Figure 7
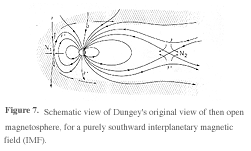
|
Figure 7 (from Hill [1983]) gives Dungey's original scenario, which assumed a purely southward directed interplanetary magnetic field (IMF). Lines 1 and 1' merge at a sunward X-type point (mp marks part of the magnetopause) to produce open lines 2 and 2', carried tailward by the solar wind in which they are embedded, to positions such as 3 and 3'. Ultimately these field lines reconnect at a distant neutral point in the tail, to produce an interplanetary field line 4 which is carried away by the solar wind, and a "closed" line 4' attached to Earth at both ends, which then flows sunward in the third dimension until it becomes the closed field line 1' which reconnects with 1. It is often held that these points are broadened to neutral lines of finite length in the direction perpendicular to the drawing, to accomodate the finite rate of flux reconnection.
The polar convection pattern and the polar electric field resulting from this motion qualitatively resemble those expected from the viscous-like drag proposed by Axford and Hines, The magnetopause now is no longer a surface containing field lines, but instead is often identified with an observed sharp discontinuity in the magnetic field, interpreted theoretically as a shock transition related to magnetic reconnection. It will have a normal magnetic component Bn which, by all predictions, is quite small (about 0.5-1 nT), making it difficult to confirm or refute this scenario by in-situ magnetic observations.
Figure 8
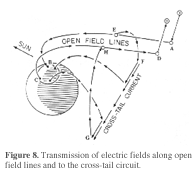
| Reconnection at N1 probably imparts little energy to the plasma. Its real significance is the creation of "open" field lines such as 2 and 2', linked to both the solar wind and the ionosphere. Because electric currents in a plasma flow easily along field lines, such lines can form a dynamo circuit, a closed circuit part of which traverses a medium moving relative to the rest. A circuit of this kind (ABCD in Figure 8) can drive an electric current and produce an electric field in the polar ionosphere: its energy is obtained by slowing down the moving solar wind or mantle plasma threaded by it, and much of that energy is then deposited as ohmic heat in the ionospheric part of the circuit.
|
Note that the currents in this circuit (in both polar caps) flow earthward on the morning side of the pole (AB) and away from Earth on the evening side (CD), which is also the pattern of region 1 Birkeland currents (below). If the tail current follows the "theta pattern" of Axford et al. [1965; Dessler and Juday, 1965], the circuit EFGH may also be viewed as a dynamo, supplying energy that heats the plasma sheet.
8. Observational Tests
Dungey's process is expected to operate best if the IMF is purely southward, for then the IMF direction matches that of the Earth's polar field lines which link up with it (Figure 9a). If the Bz component of the IMF is southward (negative) but additional components also exist, the situation is known as "southward IMF": the linkage is still relatively easy, but interplanetary field lines must bend somewhat to make the connection (Figure 9b). The bending becomes severe if Bz > 0 ("northward IMF"), because interplanetary field lines then start out headed for the "wrong" pole (Figure 9c).
| Figure 9
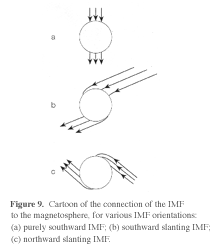
|
A major boost for Dungey's ideas was the discovery [Fairfield, 1966, 1967] that the level of magnetospheric "storminess" and of energy transfer from the solar wind to the magnetosphere depended strongly on IMF Bz. During southward IMF substorms are more frequent, the polar caps (assumed to contain open field lines) are fairly large and display a well-defined 2-cell convection pattern, and auroral ("Birkeland") currents (further below) are steady and strong. At times of northward IMF the magnetosphere is much quieter, the polar caps shrink and their E weakens. The effect is asymmetrical: when Bz is southward, increases in its magnitudes are correlated with increased activity, but the magnitude of a northward Bz seems to matter little [Burton et al., 1975]. A strong dependence of magnetospheric behavior on IMF Bz was later demonstrated by numerical simulations of the magnetosphere, conducted on fast computers and based on the MHD equations [e.g., Walker et al., 1993].
The Svalgaard effect [Svalgaard, 1968, 1972, 1973; Mansurov, 1969; Wilcox, 1972] is another interesting piece of evidence for a linkage between the IMF and terrestrial field lines. That is an asymmetry in the daily variation in polar regions, correlated with the interplanetary By component.
Owing to the interplay between solar wind outflow and the Sun's rotation, IMF field lines near Earth tend to lie close to the (x,y) plane, with B in the (x,-y) and (-x,y) quadrants and making an angle of about 450 with the x-axis, as predicted by Parker. Such lines can have one of two polarities--away from the Sun or towards it, corresponding to positive or negative By. Wilcox and Ness [1965; Wilcox, 1972] studied the prevalence of such polarities and showed that they tended to persist over times of a week or two, suggesting that the IMF in the plane of the ecliptic formed large-scale "sectors" of outward-pointing or inward-pointing field lines, co-rotating with the Sun. Often only two sectors exist, but at times they are more numerous, depending on the distribution of magnetic field sources on the Sun.
In 1926 K. Lassen established a magnetic observatory on Greenland which among other things observed the local daily magnetic variation. Around 1968 Svalgaard noted that the variation on quiet days could be classified as belonging to one of two patterns, and Wilcox suspected these correlated with interplanetary sectors. A large "blind" test was conducted [Friis-Christensen et al., 1971] and it confirmed the effect. The phenomenon might be connected with the By-related asymmetry in the pattern of polar E, later found by Heppner [1972b] ; it would be hard to explain, if terrestrial field lines had no link to the IMF.
Another observation suggesting the existence of "open" field lines is the asymmetric access to the polar caps of the high-energy tail ("strahl") of solar wind electrons (» 0.5 keV), producing the "polar rain" precipitation. Depending on the IMF sector in which the Earth is immersed, that "rain" is much more intense in the polar cap whose magnetic polarity allows direct connection to the Sun [Yaeger and Frank, 1976].
Problems in observing merging near N1 were already described. The distant nightside neutral line N2 has never been clearly identified: its signature should be a reversal of Bz from northward (Bz>0) to southward (Bz<0). Around 1983, when ISEE-3 probed the distant tail, it observed that periods of Bz<0 became more frequent at distances greater than approximately130 RE [Slavin et al., 1985], but that was only a statistical average of a rather variable quantity.
ISEE-3 has also shown [Slavin et al., 1985] and Geotail has confirmed, that in the distant tail past about 100 RE , plasma flowed tailwards at velocities that tended to increase with distance, up to where they about matched the velocity of the solar wind. This might be due to viscous transfer of plasma and momentum, but could also be the result of reconnection.
9. The Polar Aurora
Fritz [1881; Eather, 1980] estimated that, given clear skies, aurora could be observed about 100 nights a year in the region where it was most frequent. However, imaging cameras aboard satellites, more sensitive than the eye, observe a ring of diffuse aurora around the polar cap at most times. In magnetic coordinates--z along the dipole axis, the sun's direction in the x-z plane--the region where aurora is likely to occur forms a fixed pattern around the magnetic pole, known as the auroral oval [Feldshtein, 1963, 1969]. That pattern is approximately circular, centered about 5 degrees nightward of the magnetic pole [Meng et al., 1977], and the Earth rotates beneath it. The oval also expands and contracts with magnetic activity: its typical radius equals 17 degrees of latitude. The reason the aurora is a rare sight at lower latitudes is that it only appears there when the oval is grossly expanded.
For many years the identity of the primary particles producing the aurora was uncertain, although laboratory experience suggested that they behaved like cathode rays, i.e. electrons. Harang [1951, p. 140] wrote:
"It has been commonly assumed that the electrically charged particles producing the aurorae are cathode-rays, although no definite proof of this hypothesis can be given. The possibility of positive rays, a rays or protons being the primary cause of aurorae cannot be excluded."
Harang observed that the aurora penetrated to altitudes of 95-115 km, and assuming its particles were electrons, he deduced energies of 15-30 keV. The particles were first observed directly and identified as electrons in 1954, by a Geiger counter aboard a high-altitude rocket of the University of Iowa [Meredith et al., 1955]; using data from a later rocket flight McIlwain [1960] estimated a mean auroral electron energy of 6 keV. The usual greenish-grey glow of the aurora comes from the combination of N2+ bands and the 5577 A line of oxygen, but other wavelengths are also emited: some emissions are in the ultraviolet and are often used by imaging cameras aboard spacecraft, while deep red auroras at high altitudes are produced by lower-energy electrons which excite primarily the 6300 A line of oxygen.
Global studies of auroral electrons were first conducted by an Australian associate of Van Allen, Brian O'Brien, using the University of Iowa's "Injun 1" satellite, launched in 1961 and named for the "Injun territory" in which Iowa was formed. Later Injun 3 measured the distribution of arrival directions of auroral electrons (pitch angles) and also their total energy flux, and found the latter too high to be explained by the "leaky bucket" model [O'Brien and Taylor, 1964].
Among the many satellite observations of the aurora performed since that time, the most striking ones have been produced by imaging cameras, from which a global view of the entire oval can at times be obtained. The earliest images came from the Canadian Isis 2 spacecraft, launched 1 April 1971 [Lui and Anger, 1973], and they revealed for the first time the true dimensions and significance of the diffuse aurora (below). Scientifically useful results were also obtained from military imagers aboard spacecraft of the U.S. Air Force [Pike and Whalen, 1974], especially those of the DMSP series, which continues to this day; later DMSPs also carried a variety of scientific sensors. The Dynamics Explorer satellite DE-1, launched 3 August 1981 [Hoffman, 1988], carried a particularly successful imager [Frank and Craven, 1988], and more recently, several other satellites employed imagers, in particular the two Swedish spacecraft Viking [Viking Science Team, 1986; Hultqvist, 1987] and Freja, and also the Japanese Akebono [Tsuruda and Oya, 1991].
Different types of aurora may be distinguished. The brightest auroras are discrete arcs and bands. Their structure may include multiple parallel curtains, folds ("striations") and swirls of various sizes [Hallinan, 1976]. A typical electron energy spectrum in the discrete aurora [Boyd, 1975], observed above the atmosphere (Figure 10), has a peak around 5 keV and falls off steeply around 10-15 keV, while below 1 keV a large population of secondary electrons seems to exist. During substorms (sections 12-14 below) the aurora greatly intensifies and the region of such arcs expands equatorwards and intermittently polewards. The poleward expansions are highly dependent on local time and on the phase of the substorm.
| Figure 10
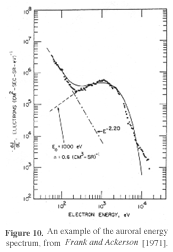
|
The diffuse aurora is fainter (detectable on the ground by photometers but not usually by the eye) and it tends to extend around the entire auroral oval; its significance was only realized after its global configuration was seen by Isis 2 [Lui and Anger, 1973]. It appears to be produced by electrons of the plasma sheet (typical energy, 1 keV) scattered into orbits that intercept the atmosphere. A mid-latitude red aurora [Rees and Roble, 1975], produced by low-energy electrons from the ring current, is usually subvisual and was discovered by Barbier [1958]. A red aurora also appears in the regions linked to the polar cusps [Shepherd, 1979], caused by magnetosheath electrons that reach the ionosphere.
"Sun-aligned arcs" appear during northward IMF and extend from the auroral oval into the polar cap, pointing roughly sunwards. They were observed by Gustafsson [1967], who felt that they were part of the regular pattern at high latitudes, rather than a separate branch; later they were studied by the Isis 2 imager [Ismail et al., 1977] and were found to be associated with low activity and northward IMF [Burch et al., 1979]. Sometimes they stretch completely across the polar cap, forming a "theta aurora" [Frank, 1986], so called because the combined pattern of the auroral oval and the arc across its middle resembles the letter theta. No generally accepted explanation of these phenomena exists and in general, the behavior of the magnetosphere during prolonged northward IMF is still poorly understood, although some interesting convection patterns in the distant tail, during such times, were recently noted by Nishida et al. [1995].
10. Field Aligned Voltage Drops
Before the spaceflight era it was often held that auroral particles came from the Sun (see BH-1). Satellite observations suggested that the acceleration process took place in the magnetosphere, but until 1974-77 it was widely believed that for electrons of auroral arcs, the most conspicuous and energetic type, this happened far from Earth, probably in the plasma sheet. Then Evans [1974, 1976a, b] proposed that the electrons which produced auroral arcs received much of their energy from field aligned voltage drops within 1-2 RE of Earth. Evidence from the S3-3 satellite (below) soon convinced the community that this indeed was the case.
Previously, many theorists believed that field-aligned voltage drops (a "parallel electric field" E//) were unimportant in magnetospheric physics, because electrons and ions moving along field lines would immediately cancel any electric charges that produced such drops. In many plasma situations, this indeed holds true. However, Alfven [1963] and his student Persson [1963, 1966] argued that E// could exist if it was balanced by the "mirror force" opposing the entry of charged particles into regions of converging field lines. Such a possibility was also known in laboratory plasma physics [Grad,1966] and is behind the operation of plasma containment machines of the tandem mirror type.
The Alfven-Persson solution will not persist in the magnetosphere under static conditions, without a constant input of energy. However, observations indicate a strong correlation between discrete arcs, where acceleration often occurs, and field aligned Birkeland currents (next section). On a distended field line, the bundle of orbits that reaches the ionosphere ("loss cone") may be too small to carry the line's share of the Birkeland current, and under such conditions, the existence of E// widens the loss cone and increases the line's capacity to carry current [Knight, 1973; Chiu and Schulz, 1978]. It is thought that such lines appropriate part of the voltage of the Birkeland circuit to provide them with the necessary E//.
An important feature of the Alfven-Persson theory is that the field aligned potential F is proportional to the intensity B of the magnetic field. A dipole field weakens with distance like r-3, hence B drops by 7/8 of its value within 1 RE of the Earth's surface, and the theory therefore predicts that the main drop of F should also occur close to Earth. As will be seen, the appearance of E// seems to be associated with field aligned currents (further below). Some theorists have also suggested that E// may arise from an "anomalous resistivity" along magnetic field lines, produced by plasma wave instabilities affecting field-aligned currents [Papadopoulos, 1977]; such processes, too, favor low altitudes where such currents have their highest density. Plasma wave instabilities are probably the source of the intense auroral kilometric radiation (AKR), discovered by Gurnett [1974]. AKR was detected before that by the first Radio Astronomy Explorer RAE-1 [Stone, 1969], but its nature and source were not recognized and the only consequence was a decision to place the follow-up satellite RAE-2 in an orbit around the moon, away from the interfering noise.
Evans [1974] proposed that many of the low-energy electrons in discrete arcs were secondaries from collisions, temporarily trapped, unable to reach the dense atmosphere below because of a magnetic mirror and unable to escape along field lines because of E// . Very clear evidence came from the S3-3 spacecraft of the US Air Force, supported by the Office of Naval Research (ONR), which detected beams of O+ ions (the dominant positive ion in the ionosphere) rising upwards, apparently impelled by the same E// which accelerated electrons downwards [Shelley et al., 1976; Johnson, 1979; Mizera et al., 1981]. In addition to the O+ beams, "ion conics" were found; these were events in which the O+ flux peaked at some intermediate angle to the magnetic field direction, suggesting that plasma wave phenomena at some lower altitude had preferrentially accelerated the velocity component v-perp perpendicular to the magnetic field [Sharp et al., 1977]. The observation of beams and conics solved the riddle of O+ ions in the ring current, first detected by Shelley et al. [1972; Sharp et al., 1974].
An alternative acceleration process, promoted by Alfven [Brush, 1990] and by Block [1972, 1978; Goertz, 1979], centered on the existence of a "double layer," an abrupt field-aligned voltage jump of appreciable intensity. Large impulsive electric fields were observed by electric field probes aboard S3-3 [Mozer et al., 1977] and the suggestion was made that they might be the signature of double layers. However, other possible explanations also exist, and no compelling evidence for the existence of such layers in space has surfaced since then.
11. Birkeland currents
Magnetic variations observed on the ground in the auroral zone are much larger than those at middle and low latitudes: swings of 500-1000 nT at auroral latitudes (out of about 60,000 nT) are much more common than 100 nT disturbances at the equator, which would be classified as fair-sized magnetic storms (Rufenach et al. [1992], Figures 12 and 13, respectively). The strong polar disturbances are localized, suggesting that the currents producing them flow nearby, probably in the ionosphere.
Birkeland [1908, 1913; Bostrom, 1968; Stern, 1977; see also BH-1] noted that the direction of the disturbance field in the auroral zone tended to be perpendicular to auroral arcs. He concluded that large electric currents flowed lengthwise along the arcs, and speculated that those currents arrived along magnetic field lines at one end of the arc and returned to space by a similar route at the other end. An overall pattern inferred in this way was later mapped, especially by Silsbee and Vestine [1942], and its currents were named auroral electrojets; they seemed to originate on the day side and to flow towards midnight along both sides of the auroral oval. Sugiura and Davis [1966] combined the readings of about a dozen magnetic observatories around the auroral zone and extracted an "AE (auroral electrojet) index" which gauged the strength of the electrojets. Values of this index are now regularly compiled and often serve as indicators of substorms and of the level of magnetospheric agitation [Rostoker, 1972b; Mayaud,1980].
Because the ionosphere conducts electricity, the existence of a dawn-to-dusk polar electric field (Figure 5b, contours viewed as electric equipotentials) suggests that an electric current flows across the polar cap; the current might enter on the morning side of the polar cap and exit in symmetric fashion on the evening side, like the current in Figure 8. The pattern of E, however, also has fringe fields that extend equatorward of the oval, to field lines that are shorter and therefore thread parts of the magnetosphere closer to Earth. In a static electric field E = -grad V, if E// is negligible, it follows from (1) that B.grad V= 0 and hence that the electric potential V is constant along field lines. The fringe pattern then maps F and E to the near-earth magnetosphere.
Schield et al. [1969] deduced from this an important new effect. The earthward flow in the tail predicted by both convection theories (Axford-Hines and Dungey) is associated (by (1)) with a dawn-to-dusk electric field E across the tail, which then maps along field lines to the polar cap, and the polar fringe pattern extends this E to nightside equatorial regions closer to the Earth. When convecting ions and electrons arrive near Earth, appreciable guiding-center drifts caused by the dipole-like internal field are added to their convective flow. These deflect the flow around the inner part of the magnetosphere, as was assumed by Axford and Hines [1961] and as was claimed even earlier by Alfven [1939; see BH-1] .
However, the magnetic drifts move positive ions and electrons in opposite directions. Schield et al. [1969] showed that as a result, if such drifts are added to the convective flow, the plasma no longer stays electrically neutral. This cannot be allowed to happen, because even a relatively tiny deviation from strict neutrality produces huge electric fields. The process may be halted in one of two ways: either E is modified in a way that keeps the plasma flow out of the region of strong magnetic drifts, or else electric currents arise along magnetic field lines (the easy flow direction in a plasma) and drain away the excess of electric charge. Both processes seem to occur.
The modification of E takes the form of "shielding," of an exclusion of E from the vicinity of the Earth, making the fringe-pattern in Figure (5b) narrower than what a calculation based solely on ionospheric conductivity would give. This was first calculated by Vasyliunas [1970] and also by Jaggi and Wolf [1973], who devised a way of simulating the process on a computer. The method was later expanded by Wolf and his group at Rice University in Houston with the "Rice Convection Model" (RCM) which simulates both the shielding and the neutralizing currents [Spiro and Wolf, 1984].
The existence of neutralizing field-aligned currents was predicted by Schield et al. [1969]. Because of Birkeland's early ideas on field-aligned currents flowing in and out of the ionosphere, they named all such currents (including the primary ones) "Birkeland currents." The neutralizing currents flow in the opposite direction from the primary currents--out of the ionosphere on the morning side and into it on the evening side, and they were expected to intersect the ionosphere somewhat equatorward of the primary currents (Figure 11). This qualitative theory was given a mathematical expression by Vasyliunas [1972].
| Figure 11
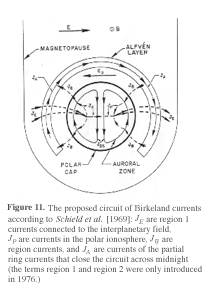
|
Current patterns like those predicted by Schield et al. [1969] were ultimately discovered by Zmuda and Armstrong [1974], using a magnetometer aboard a low-altitude satellite in polar orbit, and this, rather than flow across the pole, turned out to be the main mode by which the currents closed. Two factors delayed that discovery. The OGO-2, OGO-4 and OGO-6 missions, launched in 1965, 1967 and 1969, respectively, followed low-altitude polar orbits and carried precise magnetometers, but these instruments were intended for a survey of the Earth's internal field [e.g. Langel, 1974], and they only returned the intensity |B|, much easier to obtain accurately than the direction of B. Unfortunately, the signature of Birkeland currents is a rotation of the observed vector of B when the satellite crosses the current sheet, accompanied by practically no change in intensity. Thus the polar OGOs ("POGOs") failed to detect any field-aligned currents; later Sugiura [1975] deduced the currents' existence by observing B on near-earth passes of OGO-5, but his work appeared after the article of Zmuda and Armstrong. Some earlier observations [e.g. Zmuda et al., 1966] were also tentatively identified as signatures of field-aligned curents [Cummings and Dessler, 1967], but no global pattern was deduced.
Figure 12
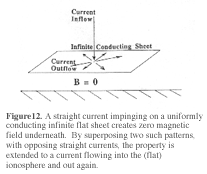
| Another delaying factor was the fact that the current flows expected from the convection pattern of Figure (5b) were quite different from the auroral electrojets inferred from ground data. One reason for the discrepancy was found by Fukushima [1969] who pointed out that when a current (Figure 12) flowed into an infinite plane conducting sheet through a perpendicular straight wire, and flowed out again by a similar wire at another point, no magnetic effect existed on the other side of the sheet. The result was later extended to a spherical geometry [Fukushima, 1976], and while the actual structure of the Earth's magnetosphere differs from these ideal cases, these results strongly suggested that Birkeland currents flowing into the ionosphere from space, across it and then out again, produced only small magnetic effects on the ground and were virtually invisible from the ground. The disturbance on the ground is almost entirely due to the auroral electrojets (further below).
|
The magnetometer used by Alfred Zmuda and James Armstrong, of the Johns Hopkins U. Applied Physics Lab, was a relatively crude instrument (resolution 12 nT), flown as an additional "piggyback" payload aboard the Navy's navigational satellite Triad. The magnetometer had no boom to keep it away from interference and it used no tape recorder, while the satellite itself--a long structure, three parts linked by long booms--swung back and forth like a pendulum.
Yet Triad observed very clearly the predicted rotations of B. Zmuda and Armstrong found two parallel current sheets following the morningside auroral oval for almost its full length, with the polar sheet flowing into the ionosphere and the equatorial one out of it; two similar sheets, but with opposite flow directions, were found along the eveningside oval. At the suggestion of Masahisa Sugiura, Iijima and Potemra [1976a, b] later named the polar sheets "region 1" and the equatorward ones "region 2" (Figure 13). Tragically, by the time their work was published [Zmuda and Armstrong, 1974], both authors had died--Zmuda of an untimely heart attack, Armstrong by suicide.
| Figure 13
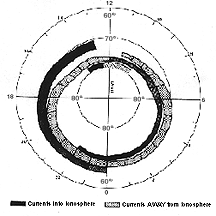
|
Figure 13. A map of the polar ionosphere, showing the average configuration of Birkeland (field-aligned) currents there. Regions where the current enters the ionosphere have dark shading, regions where it flows away from Earth and into space have light shading. From Iijima and Potemra, [1976b]; the origin is at the magnetic pole and the Sun's direction is on top.
About 75% (the proportion varies) of the current reaching Earth in region 1 leaves again as region 2, while the rest closes across the polar cap or around the auroral oval. The flow through the ionosphere encounters an anisotropic conductivity [Cowling, 1945; Dungey, 1958; Bostrom, 1964; see also e.g. Kelley, 1989]: in addition to a "Pedersen" current density in the direction of E, there also exists a "Hall" current density at right angles, of comparable or larger magnitude. The concentrated electrojet is largely the Hall current associated with the linkage between the systems of regions 1 and 2 across the ionospheric gap between them, although it may also include Pedersen currents, guided along the auroral oval by a channel of higher conductivity due to precipitation of auroral electrons. As for the portion of the current flowing across the polar cap, it may be unevenly divided between the two hemispheres, especially near solstice when the sunlit summer ionosphere conducts far better than the dark winter ionosphere, leading to a seasonal effect discovered by Fujii et al. [1981].
The pattern of Figure 13 was derived from Triad observations by Iijima and Potemra [1976a, b] and is marked by lines of magnetic local time (MLT), measured around the magnetic pole with noon in the Sun's direction. Note that the transition between the ingoing and outgoing portions of the pattern is centered not at midnight but around 2200 MLT: a similar rotation of the electric field pattern should be added in Figure (5b) and could be due to the Hall conductivity [Vasyliunas, 1970]. The crossing-over beginning near 2200 MLT coincides with the region of changes in auroral and magnetic activity known as the Harang discontinuity Heppner [1972b; Fukushima, 1994, appendix D]. Overlaps exist at midnight and additional currents are observed near noon, possibly associated with the cusps; some have named them "region zero.".
It should be stressed that Figure 13 is a statistical average and that actual sheets are much more fragmented and irregular (e.g. Plate 2 of Bythrow et al., [1984]). The greatest intensity of region 1 currents occurs on the day side, in agreement with the observation [Heelis et al., 1976] that E, too, is strongest in a "throat region" near noon. During substorms region 1 is reinforced by a "wedge current" diverting part of the cross-tail current earthward [McPherron et al., 1973]; during northward IMF Bz, the system may weaken and almost disappear [Rich and Gussenhoven, 1987] but characteristic "NBZ currents" may then be observed on the day side, strongly dependent on IMF By and possibly related to the Svalgaard effect.
The flow of region 1 currents far from Earth is still being debated. While some currents near noon may flow down directly along open field lines in the manner of circuit ABCD in Figure 8, the tracing of polar field lines using data-based models of the magnetic field suggests that most of them flow on closed field lines [Stern, 1992] and may therefore be connected to the cross-tail current and to its sunward extensions (Atkinson [1978], Figure 3). Most recently Tsyganenko et al. [1993] found in-situ statistical evidence suggesting that a significant part of the nightside region 1 flow, on the nightside, originates in the plasma sheet, at distances of 10-30 RE, with very little coming from greater distances.
12. Substorms: early observations
Following a great auroral display in Connecticut, on July 1, 1837, E.C. Herrick [1838, cited by Siscoe, 1980] wrote (italics in the original):
"It is worthy of notice that on this occasion there were two well marked and distinct seasons of greatest brilliance or fits of maximum intensity, at intervals of about four hours. It will be found on examination of former accounts, that this is a common feature of Auroral exhibitions of unusual brilliance."
Birkeland [1908, 1913; Bostrom, 1968;] observed conspicuous magnetic signatures of such "fits of maximum intensity" and proposed that here was a new type of magnetic activity, an "polar elementary magnetic storm" with a typical time scale of half an hour. More about Birkeland's pioneering work is given in BH-1 and its references, and also in the work of Stern [1991], from which parts of this section are taken.
Birkeland's polar storms are now known as (magnetic or magnetospheric) substorms. These violent twitches of the Earth's magnetic tail energize ions and electrons, inject some of them into the ring current, and greatly increase the rate at which energy is released in the magnetosphere. Indeed, many parallels exist between substorms and impulsive particle acceleration events on the Sun, and both are believed to be powered by the conversion of magnetic field energy. For all these reasons the substorm may be the most interesting problem in magnetospheric physics and a great challenge to both observer and theorist.
As noted in BH-1, Birkeland's ideas were opposed by Sidney Chapman [Chapman and Bartels, 1940; Fukushima, 1994, sect. 6]. Chapman contrasted the short time scale of polar disturbances with the much longer one of global magnetic storms and proposed that "polar storms" were merely phases of the global storm. Around the middle of the century such events were called "magnetic bays" because on a magnetogram (the plotted output of a magnetic observatory) they resembled bays on a coastline (according to Chapman and Bartels [1940], this term is due to Chree [1911,1912]). A study of bays was conducted by Silsbee and Vestine [1942; also Fig. 2 , Stern, 1977] who deduced a 2-cell pattern with strong electrojets near the boundary of the polar cap.
While Birkeland believed that auroral currents originated in distant space, the consensus in mid-century was that they resembled the well-known diurnal magnetic variation whose currents were attributed to tidal dynamo effects in the ionosphere, and that they were completely contained inside the ionosphere [Vestine and Chapman, 1938]. It was thought that the large polar magnetic variations arose by a similar process, but were much more intense because ionospheric conductivity was enhanced in regions bombarded by the aurora. Thus Harang [1951; p. 94] wrote, after explaining the tidal dynamo:
"The intrusion of electrically charged particles which produce the aurorae, strongly increases the ionisation and thus the conductivity of the ionised layers. Besides this, one must also assume secondary effects, such as expansion or heating of the upper atmosphere, which may increase the movements of the layers along the auroral zone. The polar storms are therefore, according to these views, due to an increase in the conductivity and velocity of movements of the upper layers."
Chapman became more involved with the aurora after 1951, when he accepted a visiting professorship at the University of Alaska at Fairbanks, and after he retired from Oxford in 1953 he used to stay in Fairbanks several months each year [Akasofu, 1970]. In 1958 he was joined there by Syun-Ichi Akasofu, a young Japanese who became his main associate.
The word "substorm" first appeared in Chapman's writings in 1961 [Akasofu and Chapman, 1961, p. 1339], referring to a bay-like disturbance assumed to be a phase of the global magnetic storm. Two years later Akasofu and Chapman [1963] compared the signatures of magnetic storms near the equator and in the auroral zone (Figure 14). Near the equator the magnetic field variation was simple and familiar, a gradual weakening of the field on a typical time scale of 6 hours, followed by a slow recovery. In the auroral zone, on the other hand, the magnetic record was punctuated by many short but intense magnetic bays, which the authors again named substorms.
| Figure 14
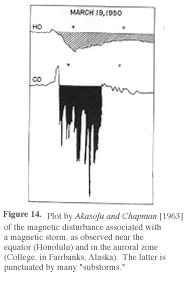
|
13. Substorms: the Satellite Era
The International Geophysical Year (IGY) 1957-8 (actually extended to a year and a half) brought not only the first scientific satellites but also a great expansion in auroral observations and widespread use of "all-sky cameras" [see Eather, 1980] which photographed the entire sky as reflected in a convex mirror. Using such records, Akasofu noted in 1964 that magnetic bays, which also occurred widely outside magnetic storms, were associated with a distinct pattern of auroral intensification and expansion, and proposed to name the phenomenon "auroral activation" [Akasofu, 1970]. Chapman however insisted on "auroral substorm" and that was the name used in the article's title [Akasofu, 1964]. Later Akasofu favored "magnetospheric substorm" [Akasofu, 1977; Siscoe, 1980], while Rostoker [1972a has used "polar magnetic substorm"; the commonly used term nowadays is "magnetic substorm" or simply "substorm." Today's view is that the "substorms" which Chapman identified inside magnetic storms are of a similar nature, except perhaps bigger and more frequent, capable of injecting appreciable numbers of ions and electrons into long-lived orbits of the ring current region [Lui et al., 1987].
During 1964-6 Akasofu and his group studied the morphology of substorms in great detail [Akasofu et al., 1964, 1965a,b,c; 1966a,b,c,d; Akasofu, 1966]. Their "classical" substorm phases (individual storms may vary) are still accepted: an initial brightening of a quiet arc, the expansion of the aurora polewards (either by the motion of existing arcs or by formation of new ones), a westward surge along the auroral oval, gradual breaking-up of arcs and final recovery.
A deeper understanding was gained after about 1965, when satellites began observing the great changes accompanying substorms in the Earth's magnetic tail. They observed magnetic field lines becoming stretched prior to substorm onset (Figure 15, from Fairfield and Ness [1970]) and then rebounding to more dipole-like shapes ("dipolarizing"). This was also noted by Heppner [1967] who wrote (p. 184):
"The view that is favored is that the tail field is partially collapsing back towards a less stressed condition during a negative bay."
| Figure 15
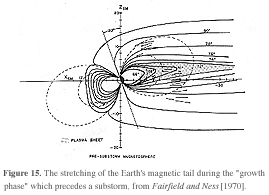
|
The preliminary stretching may be observed as close as synchronous orbit, at 6.6 RE [e.g., Baker et al., 1981] and may begin as long as one hour before substorm onset: the prevalent idea (originally widely debated) is that this is the "growth phase" during which magnetic energy is stored [McPherron, 1970, 1972], and often an analogy is drawn between the stretching of field lines in this phase and the stretching of a slingshot. When the field lines rebound, the stored energy goes to accelerate particles and to drive currents. A large increase in the flux of ions and electrons is then observed at the nightside near the inner edge of the cross-tail current, e.g. in the synchronous orbit at 6.6 RE [De Forest and McIlwain, 1971], but what exactly goes on is still not understood [Mauk and Meng, 1987]. Typical injected energies are 1-10 keV, but particles up to 100 keV and more have also been observed [Nagai et al., 1983].
Satellites in the plasma sheet may observe disappearances ("drop-outs") of the plasma at the time of onset, and after onset a satellite which had been outside the plasma sheet may be suddenly engulfed by it. Early observations of such changes [Hones et al., 1967, 1970; Hones, 1979] were complicated by the fact that they were performed by "piggyback" instruments aboard the Vela satellites which carried no magnetometers, since their primary mission was to detect violations of the ban on nuclear tests in space. The ultimate explanation was that the plasma disappeared when the plasma sheet was severely stretched and became extremly thin, while the sudden appearances of plasma were associated with dipolarization, when plasma energized by the substorm rebounded earthward, swelled field lines of the near-earth tail and extended the plasma sheet past the satellite.
The deep magnetic bays accompanying the substorm arise from greatly enhanced electrojets, and the AE index [Sugiura and Davis, 1966] is often taken as a gauge of the level of substorm activity. Observations have suggested [McPherron et al., 1973, Fig. 7-8] that during substorms Birkeland currents are reinforced on the nightside by a system with region 1 polarity, arising from a diversion of part of the cross-tail current through the ionosphere. Because the shape of the diversion circuit tapers towards the Earth, this is known as the "substorm wedge current". In the ionosphere the wedge current follows the auroral oval, whose conductivity is greatly increased by the aurora, and thus reinforces the westward electroject around midnight. The wedge current may be intense enough for its magnetic effects to be observed at middle latitudes [Clauer and McPherron, 1974a, b] as well as in synchronous orbit [McPherron and Barfield, 1980].
By 1972 most observational features of the substorm had been identified, and they were reviewed by Rostoker [1972a] who also summed up the history of substorms (p. 163, 200), and by Aubry [1972]. A conference on substorms was held in October 1972 [Vasyliunas and Wolf, 1973] and an initial coordinated study of the substorms of 15 August 1973 was undertaken. The results of that study appeared in 1973 in 9 consecutive articles (J. Geophys. Res., 78, p. 3044-3149) the last of which [McPherron et al., 1973] presented an interpretation which included the "wedge circuit."
14. Substorms: Theory
As the features of substorms became known, attempts were made to explain them. One important feature was the strong correlation between substorms and "southward IMF". If the magnetosphere was quiet during a spell of northward IMF Bz, and suddenly Bz turned southward and stayed that way, it was found that a high probability existed for a substorm to erupt within an hour or so.
A further link was provided by Aubry et al. [1970; Aubry and McPherron, 1971] who found evidence that, other things being equal, the "nose" of the magnetosphere was pushed in closer to Earth at times of southward IMF Bz, a phenomenon qualitatively evaluated by Holzer and Slavin [1978] and by Sibeck et al. [1991; Roelof and Sibeck, 1993]. Aubry termed this phenomenon "erosion" of the magnetopause and claimed it occurred because closed field lines were being reconnected to interplanetary ones near N1 (Figure 7) faster than closed lines were arriving from the tail to take their place.
Coroniti and Kennel [1972] explored later developments of this scenario. If magnetic flux is removed from N1 faster than the sunward flow initiated near N2 brings it back, additional flux will pile up in the tail lobes. The lobes then swell and present a larger obstacle to the solar wind, which therefore compresses them more, increasing the lobe field BL: they identified this process with the growth phase of substorms, noting that the magnetic energy in the tail, whose density is proportional to BL squared, would increase.
Figure 16

| Ultimately, in this scenario, the increased southward IMF reaches N2 and increases the rate of reconnection there, and after a while the supply of returning magnetic flux reaching N1 again matches the demand. But meanwhile other processes may intervene. The increased pressure on the lobe may squeeze the plasma sheet, causing reconnection at an internal neutral line N3 (Figure 16), so that the flux returning sunward is now supplied by reconnection at N3. Tailward of N3 an isolated magnetic bubble will be created, named "plasmoid" by Hones [1976; p. 567], a term previously applied by Bostick [1956, 1957, 1986] to a type of transient plasma bubble observed in the laboratory. Hones [1979, p. 393] described it as "...a blob of magnetospheric plasma ... detached from the magnetotail plasma...". The reconnection process at N3 was assumed to provide the substorm's energy and to accelerate particles; observations of impulsively accelerated particles in the tail [e.g. Keath et al., 1976; Roelof et al., 1976] were believed to indicate proximity to to N3.
|
The preceding scenario, with various modifications, has dominated the community's view of substorms in the last 20 years. In particular, Hones [1979] has collected evidence in its favor, and has argued that once a new neutral line N3 was formed, the tailward motion of the plasmoid would stretch it into a neutral sheet, explaining the formation of a very thin section of the plasma sheet ("thinning") inferred from the observed disappearance of plasma. This was disputed by Frank et al. [1976] who claimed substorms originated in "fireballs", possibly associated with boundary layers. The controversy persisted for a while [Hones, 1977, 1978a, b, c; Frank and Ackerson, 1977; Frank et al., 1978a, b], with Hones claiming that Frank's examples were in fact ordinary reconnection events, but it ultimately died down when no alternative scenario based on fireballs emerged.
Since that time several alternative theories of the substorm have been proposed [Kan, 1990]. Most interpretations place N3 fairly close to Earth (about 15 RE), but some views [Rostoker and Eastman, 1987] argued that substorms may reflect enhanced reconnection (and other processes?) at N2. Alternative theories have invoked a "thermal catastrophe" [Goertz and Smith, 1989], Alfven waves bouncing between the ionosphere and the tail [Kan et al., 1988] and disruption of the cross-tail current [advocated by Lui, 1991]. The proliferation of models has led to some skepticism [Stern, 1989b], and a meeting was held in Victoria, B.C. to seek some general agreement [Rostoker et al., 1980], but it did not clarify much.
One approach to studying substorms is to try correlate their variations with interplanetary stimuli, and thus seek to identify their causes or "triggers." Part of the problem here is the gauging of a substorm's intensity, and many studies have used for this the auroral AE index, or the related AU and AL indices. These reflect the strength of the auroral electrojets and therefore of the Birkeland current system, and they are known to become very high during large substorms.
Coroniti and Kennel [1972], Hones [1979] and most other researchers argued that the substorm obtained its energy from magnetic energy stored in the tail lobes, accumulated during a "growth phase" preceding substorm onset, during which the tail's magnetic flux increased [Caan et al., 1975]. Perreault and Akasofu [1978], however, found good correlation of AE with an "epsilon-parameter," constructed from solar wind characteristics and very sensitive to IMF Bz. They therefore proposed that substorms represented periods of stronger coupling between the solar wind and the magnetosphere, enabling the latter to extract more energy, and were thus "driven" by interplanetary conditions rather than representing the "unloading" of stored energy [Akasofu, 1980]. Some physicists now claim that both "unloading" and "driven" processes are involved. Predictive linear filters have also been used to study the way the solar wind input relates to AE [Iyemori et al., 1979; Clauer et al., 1981].
Theorists who support the "near-earth neutral line" (NENL) scenario have sought the "trigger" mechanism which initiates the onset of substorms, possibly some plasma instability. Computer simulations based on idealized MHD equations and assuming (purely) southward IMF [e.g., Walker et al., 1993] have supported the NENL scenario, yielding (with southward IMF) even more pronounced reconnection than seems to be observed.
15. Convection in the geotail
An interesting idea about the origin of substorms was proposed by Erickson and Wolf [1980; Hau et al., 1989; Erickson, 1984, 1992]. In the ideal MHD approximation, in the absence of rapid accelerations, it is expected that the magnetosphere is always close to force balance, mainly between pressure gradients and the magnetic force:
grad p= j x B (2)
Properly p is a tensor, but in the plasma sheet the observed distribution of ions is close to isotropic and hence a scalar p is often used there. Solutions of (2) appropriate to a realistic 3-dimensional magnetosphere are not known, but two-dimensional solutions for a scalar p, assuming a linear dipole that extends indefinitely in the y-direction, can be obtained (at least numerically) from the Grad-Shafranov equation [e.g. Voigt and Wolf, 1988].
Erickson and Wolf [1980] noted that the existence of a convective plasma flow in the tail imposes additional restrictions. If the tail's field lines move with the flowing plasma, either the magnetic pattern is static and satisfies (2), and then the convection is such that each field line of the pattern is carried into another one; or else the pattern evolves, in which case (2) must hold at each intermediate stage. Erickson and Wolf showed that observed patterns of B and expected patterns of E were not compatible with a static scenario.
Erickson [1992] later simulated the field's evolution on a computer, satisfying (2) at all times; his calculation was two-dimensional, but flux arriving near Earth was allowed to "escape sideways" rather than piling up. This process led to a very weak B near the earthward edge of the plasma sheet (x near -12 RE), suggesting in almost all cases the imminent formation of a near-earth neutral line (the simulation could not go far enough to confirm it). By this scenario, substorm-type events may be an inevitable outcome of convection in the tail.
Attempts to actually observe this convection raise new problems. The double-probe method for observing E in low Earth orbit fails in the rarefied plasma of the tail, but the plasma's bulk flow can be inferred by measuring ion flux anisotropies. The first attempt [Frank and Ackerson, 1979] suggested a great deal of back-and-forth sloshing of plasma, but no underlying persistent earthward motion. A later study by Huang and Frank [1986] filtered out plasma sheet boundary layer observations, which contained field-aligned flows, and obtained an average earthward flow in the plasma sheet (r < 22 RE) of about 20 km/s, much below the expected rate. Recent studies by Angelopoulos et al. [1992] suggest that high-speed earthward plasma flows do exist (at about 150 km/s) but only about 7% of the time. The problem is thus still unsolved.
16. Planetary Magnetospheres
Space missions to othe planets of the solar system have shown that most of them are magnetized. In particular, the giant planets are magnetized much more strongly than Earth [Bagenal, 1992] and their magnetospheres are all much larger than ours, in part because of the stronger dipole moments, in part because the solar wind becomes increasingly rarefied far from the Sun. Tiny Mercury has a magnetic moment only about 1/2000 that of Earth and a very small magnetosphere, Venus seems non-magnetic and Mars may or may not have a weak field. The magnitudes of the dipole moments of Mercury, Earth, Jupiter, Saturn, Uranus and Neptune, in units of 10^25 Gauss-cm3, are 0.004 (approx.), 7.9, 150,000, 4300, 420 and 200, respectively [Lepping, 1995].
The most striking thing about these magnetospheres is their great diversity and this brief overview cannot possibly do justice to the extensive research done on them. Good accounts of the initial observations and of many associated discoveries can be found in special sections of the journal Science [1974, 1975a, 1975b, 1979a, 1979b, 1980, 1981, 1982, 1986, 1989, 1992], published soon after the planetary encounters by Pioneer 10 (Jupiter), Mariner 10 (Mercury), Pioneer 11 and Voyager 1 (Jupiter and Saturn), Voyager 2 (Jupiter, Saturn, Uranus and Neptune) and Ulysses (Jupiter). The Galileo spacecraft reached Jupiter in December 1995 and entered an orbit around the planet, after successfully launching a probe into Jupiter's atmosphere.
The strongest magnetic field and the most intense trapped radiation are found in the magnetosphere of Jupiter, which is also the largest [Dessler, 1983]. This was furthermore the first planetary magnetosphere to be discovered: in 1955 strange radio noise was traced by Burke and Franklin to the planet Jupiter [Franklin, 1959, 1985], although it was only attributed to magnetically trapped plasma after the discovery of the Earth's radiation belt [Drake, 1985].
Jupiter's magnetosphere is loaded with ions of sulfur and also of sodium, ejected from "volcanoes" on the satellite Io. Io also has an ionosphere with an interesting dynamo interaction with Jupiter [Ness et al., 1979]. Jupiter's trapped plasma carries a dense ring current, and seems to co-rotate with the planet, perhaps up to the magnetopause. Its density profile contains dips due to absorption by Jupiter's moons and by the planet's thin ring, which resembles Saturn's ring but is much narrower; the existence of that ring was first suggested by an absorption feature in the belt [Acuna and Ness, 1976]. Jupiter also has an aurora, observable from Earth, and radio emissions with complicated patterns, some of them correlated with the position of Io.
Saturn's magntosphere similarly tends to rotate with the planet and contains absorption features. The planet seems to have an inner belt like the Earth's, believed to arise from albedo neutrons knocked out of the planet's rings by cosmic rays [Cooper and Simpson, 1980].
The Earth's magnetic axis is very close to its rotation axis. Similar proximity between the two axes was found for Jupiter, Saturn and Mercury (for Saturn the axes coincided within observational error), and this was therefore widely held to be a general feature of planetary magnetic fields. At the time of the encounter between Voyager 2 and Uranus, on 24 January 1986, the planet's axis pointed within a few degrees of the Sun. It was therefore expected that here was a "pole-on" magnetosphere, a previously unstudied configuration in which the axis of the planetary magnet pointed approximately into the solar wind.
But it was not to be. The magnetic axis of Uranus--and later also that of Neptune--was found to make an angle of about 60 degrees with the planetary rotation axis, causing the field to swing widely with each rotation of the planet. As Uranus orbits the Sun, there will arise occasions when a "pole-on" magnetosphere is (briefly!) realized, but it did not happen during the Voyager 2 encounter.
Finally, Mercury's magnetosphere [Ness, 1979] seems to be too small for energetic particles to become trapped in it. However, as Mariner 10 went past the planet's night side, it encountered a burst of energetic particles, which could be the result of a substorm-type event in Marcury's magnetic tail.
Interesting magnetic cavities are also formed around Venus, the Moon and comets (and probably, Mars), but if the obstacle is not a planetary magnetic field, the cavity produced is quite different from the ones described above. All this suggests a rather rich field for future research, involving configurations unlike the Earth's, on which many additional observations still remain to be made.
17. Other Areas
A brief overview like this one must by necessity omit many important topics, such as:
- (1) Instrumentation:
- e.g. magnetometers [Heppner, 1963; Ness, 1970], electric field probes [Fahleson, 1967; Cauffman and Gurnett, 1972]; charged particle detectors and mass spectrometers. A sampling of articles or collection of articles (only first paper cited) on specific spacecraft and their instruments includes Injun 3 [O'Brien et al., 1964], the OGO series [Ludwig, 1963; Bostrom and Ludwig, 1966], Atmosphere Explorer 1 [Dalgarno et al., 1973], S3 or Explorer 45 [Longanecker and Hoffman, 1973], International Sun-Earth Explorer (ISEE) 1 and 2 [Ogilvie et al., 1978], Active Magnetospheric Particle Tracer Experiment (AMPTE) [Acuna et al., 1985] and Dynamics Explorer (DE) 1 and 2 [Hoffman et al., 1981]
- (2) Wave phenomena in the magnetosphere:
- [Shawhan, 1979], including whistlers [Helliwell, 1965; Al'pert, 1980; see also BH-1], auroral kilometric radiation [Gurnett, 1974], micropulsations [Hughes, 1983; Lanzerotti and Southwood 1979], 3/2 cyclotron frequency emissions, auroral hiss and other modes.
- (3) The bow shock of the Earth:
- [Dobrowolny and Formisano, 1973; Greenstadt and Fredricks, 1979; Kennel et al., 1985; Kennel, 1987].
It is hoped that scientists and historians familiar with those areas will add their histories to the record.
18. Assessment
The preceding brief history only covers scientific aspects of magnetospheric physics. In addition, magnetospheric physics also has institutional, personal and social aspects.
An institutional history traces the evolution of the field and its accomplishments in the framework of the organizations which led it, of institutions, committees, executive decisions and of the individuals involved in them [e.g. Ezell, 1988]. An instructive example is "Beyond the Atmosphere" [Newell, 1980], an account of NASA's effort in space science 1958-1975 by a former NASA Associate Administrator who led those efforts for many years. It covers all fields, not just magnetospheric physics, but where its subject overlaps this narrative, it often paints a strikingly different picture.
Personal histories are first-hand accounts by participants. At best they give an unequalled intimate view of the discovery process. At worst they are carefully filtered, and their writers also do not always have the necessary discrimination and writing skill. Such deficiencies would matter less if such accounts were plentiful enough to allow comparison and cross-checking: sadly, only very few exist, which makes them particularly valuable, and their coverage of the field is rather patchy [Van Allen, 1983a, 1990 ; Eather, 1980, chapt. 19; Frank, 1990; Gombosi et al., 1994].
The community of magnetospheric physics has never been properly studied. It is relatively small: the membership of AGU's Section on Space Physics and Aeronomy stands around 3000 (1980--1604; 1985--1922; 1990--about 2600). This also includes scientists whose main interests are the upper atmosphere, interplanetary space and the Sun, but on the other hand may miss many workers outside the USA. As noted, this discipline arose from three main sources--plasma physics, work with rockets, balloons and ground instruments, and the study of cosmic rays. It began assuming its separate identity in 1959, when (led by James Van Allen) it chose the American Geophysical Union (AGU) as its home organization and the Journal of Geophysics Research (JGR) as its main means of communication.
Today that community is in a serious crisis, made evident, for instance, by a frustrating slow-down in the rate of discovery during the last decade 1984-1994. It may be instructive to speculate about the causes of this slow-down and its implications to the community's future.
One can roughly divide the record of magnetospheric physics in the space age into three periods: (1) the era of discovery, 1958-1965; (2) the expansion stage, 1965-1977; and (3) the era of stagnation, setting in gradually after 1977.
In the first period, the large-scale morphology was surveyed--particle populations, the main regions and the boundaries. In addition, this was the beginning of our ideas on convection and reconnection.
In the expansion stage, details were filled in--correlations with the IMF, substorm morphology, Birkeland currents, E//, auroral kilometric radiation, O+ ions in the ring current, ion beams and conics, injections at synchronous orbits, etc. Additional theoretical ideas were also introduced--the NENL theory of substorms, the Brice-Nishida theory, the Coroniti-Kennel theory, theories on the consequences of convection by Schield et al. and by Vasyliunas, and others not touched on here.
Since 1977 some observational details were added., e.g., about the magnetosphere with IMF Bz > 0, about the distant tail (by ISEE-3 and Geotail), the ring current (by AMPTE-CCE) and the plasma sheet (by ISEE 1-2 and AMPTE-IRM). Theories, too, have improved, but the main problems continue to elude us--the nature of substorms, structure of the open magnetopause, specifics of reconnection, convection in the tail, global structure during northward IMF and similar questions.
Why this apparent pause? Three possible reasons will be noted here: the nature of discovery, the choice of mission strategy and a missed transition in the evolution of magnetospheric physics.
(1) There exist two kinds of discovery in this field--discovery of new problems and discovery of solutions. The heady early period seemed packed with discoveries, but most of them belonged to the first kind. It was inevitable that satelites passing for the first time through the radiation belt, the magnetopause, cusp, bow shock or plasma sheet would make an important discovery; but while new phenomena accumulated, explanations of their features lagged, and they still do. In laboratory physics, when a new phenomenon is discovered, one can design experiments to focus on it; but magnetospheric physics, in common with the rest of geophysics, offers few controlled experiments and depends primarily on observations. Thus progress towards explanations is slow and uncertain.
(2) The cost of spacecraft is high, both in funds and efforts. All early space missions therefore involved isolated spacecraft, but it seems that the amount of information available from this mode is just about exhausted. Magnetospheric physics is synergistic: to understand global behavior, a coordinated network of satellites is needed.
After 1977 the field was ripe for such a network, but unfortunately the use of isolated spacecraft is still the norm. The Russian Interball (two spacecraft launched in 1995) and the European Cluster (due in 1996) each contain four coordinated spacecraft and promise to give valuable results, in particular in conjunction with the "Wind" and "Polar" spacecraft of the US. But a meaningful coverage demands a much larger number of platforms, as was made clear by CDAWs, Coordinated Data Analysis Workshops [e.g. Manka et al., 1982], which tried to analyze specific events and generally found that even with all available data, important questions could not be resolved.
(3) As noted, the magnetospheric community first assumed a separate identity around 1960. Independent space physics departments were established at selected universities--Iowa, UCLA, Rice, Alaska, then more--and space research groups were set up at NASA, Johns Hopkins Applied Physics Lab., Los Alamos etc. As the community expanded in 1965-77, it also began raising its first generation of internally-trained scientists.
But something seemed missing. A community needs not only its institutional identity but also a core of its accumulated knowledge, set up in an orderly way that can be passed on. Research and symposia lead to review talks and papers, which in turn lead to textbooks and courses, telling "such-and-such we are pretty sure of and can teach, this-or-that is unclear or controversial, and here are the boundaries of our knowledge."
Even now, rather little of this process of distillation has taken place, especially in observations: in substorm morphology, for instance, there is surprisingly little that can be regarded well-established. Possible reasons are too long and too controversial to list here, but the result has been a narrowness of scope and a lack of broad vision which even now hamper further progress and further planning.
This review is altogether too short to properly describe what such "core knowledge" may contain. Still, one hopes it will give its readers, especially younger members of the community, a uniform historical framework of the overall structure of their field.
Acknowledgments
A draft of this article was given for review to a large number of scientists involved in the discoveries described here, most of whom provided useful comments. The author thanks A. Dessler, J. Heppner, P. Hart, W. Hess, R. Hoffman, E. Hones Jr., G. Ludwig, M. Peredo, H. Petschek, J. Slavin, E. Smith, J. Van Allen, M. Walt and R. Wolf for their help in compiling this review, as well as two editors and three referees of the journal.
References
Note: Journals are cited by their most recent name; for instance, "Reviews of Geophysics" was "Reviews of Geophysics and Space Physics" in 1970-1984. The mark (c) indicates the first in a collection of articles.
- Acuna, Mario H. and N.F. Ness
- Summary of the initial results from the GSFC fluxgate magnetometer on Pioneer 11, in Jupiter, pp. 830-847, edited by T. Gehrels, U. of Arizona Press, 1976.
- Acuna, Mario H., G.W. Ousley, T.W. McEntire, D.A. Bryant and G. Paschmann (c)
- AMPTE mission, overview, IEEE Trans. Geoscience Electr., GE-23, 175-176, 1985
- Aggson, Thomas L. and J.P. Heppner
- "Probe measurements of electric fields in space", in Atmospheric Emissions, pp. 305-316, B.M.McCormac editor, Van Nostrand Reinhold, New York 1968.
- Akasofu, Syun-Ichi
- The development of the auroral substorm, Planet. Space Sci.,12, 273-282, 1964.
- Akasofu, Syun-Ichi
- The development of geomagnetic and auroral storms, J. Geomag. Geoelect., 18, 109-123, 1966.
- Akasofu, Syun-Ichi
- In memoriam Sydney Chapman, Space Science Reviews, 11, 599-606, 1970.
- Akasofu, Syun-Ichi
- Physics of Magnetospheric Substorms, xviii + 599 pp., D. Reidel, Dordrecht, 1977.
- Akasofu, Syun-Ichi
- The solar wind-magnetosphere energy coupling and magnetosphere disturbances, Planet. Space Sci., 28, 495-509, 1980.
- Akasofu, Syun-Ichi and Sydney Chapman
- The ring current, geomagnetic disturbance and the Van Allen radiation belts, J. Geophys. Res., 66, 1321-50, 1961.
- Akasofu, Syun-Ichi and Sidney Chapman
- The development of the main phase of magnetic storms, J. Geophys. Res., 68, 125-129, 1963.
- Akasofu, Syun-Ichi, D.S. Kimball and Ching-I. Meng
- The dynamics of the Aurora, I, Instabilities of the aurora, J. Atmos. Terr. Phys., 26, 205-211, 1964.
- Akasofu, Syun-Ichi, Sidney Chapman and Ching.-I. Meng
- The polar electrojet, J. Atmos. Terr. Phys., 27, 1275-1305, 1965a.
- Akasofu, Syun-Ichi, D.S. Kimball and Ching-I. Meng
- The dynamics of the Aurora, II, Westward traveling surges, J. Atmos. Terr. Phys., 27, 173-187 1965b.
- Akasofu, Syun-Ichi, D.S. Kimball and Ching-I. Meng
- The dynamics of the Aurora, III, Westward drifting loops, J. Atmos. Terr. Phys., 27, 189-196, 1965c.
- Akasofu, Syun-Ichi, D.S. Kimball and Ching-I. Meng
- Dynamics of the Aurora, IV, Polar magnetic substorms and westward traveling surges, J. Atmos. Terr. Phys., 28, 489-496, 1966a.
- Akasofu, Syun-Ichi, D.S. Kimball and Ching-I. Meng
- Dynamics of the Aurora, V, Poleward Motions, J. Atmos. Terr. Phys., 28, 497-503, 1966b.
- Akasofu, Syun-Ichi, D.S. Kimball and Ching-I. Meng
- Dynamics of the Aurora, VI, Formation of patches and their eastward motion, J. Atmos. Terr. Phys., 28, 505-511, 1966c.
- Akasofu, Syun-Ichi, D.S. Kimball and Ching-I. Meng
- Dynamics of the Aurora, VII, Equatorward motions and the multiplicity of auroral arcs, J. Atmos. Terr. Phys., 28, 627-635, 1966d.
- Alfven, Hannes
- A theory of magnetic storms and of the aurorae, K. Sven. Vetenskapakad., Handl., Ser. 3, 18(3), 1939. (Reprinted in part with comments by Alex Dessler and John Wilcox in Eos, 51, 180-194, 1970).
- Alfven, Hannes
- Cosmical Electrodynamics, Oxford University Press, 1950.
- Alfven, Hannes and Carl-Gunne Falthammar
- Cosmical Electrodynamics, Fundamental Principles, Oxford University Press, 1963.
- Alpert, Yakov
- 40 years of Whistlers, J. Atmos. Terr. Phys., 42, 1-20, 1980.
- Angelopoulos, Vassilis, W. Baumjohann, C.F. Kennel. F.V. Coroniti, M.G. Kivelson, R. Pellat, R.J. Walker, H. LŸhr and G. Paschmann
- Bursty bulk flows in the inner central plasma sheet, J. Geophys. Res., 97, 4027-4039, 1992.
- Atkinson, Gerald
- Energy flow and closure of current systems in the magnetosphere, J. Geophys. Res., 83, 1089-1103, 1978.
- Aubry, Michel P.
- A short review of magnetospheric substorms,p. 357-364 in Earth Magnetospheric Processes, Billy McCormac, ed., D. Reidel, 1972.
- Aubry, Michel P., C.T. Russell and M.G. Kivelson
- Inward motion of the magnetopause before a substorm, J. Geophys. Res., 75, 7018-7031, 1970.
- Aubry, Michel P. and Robert L. McPherron
- Magnetotail changes in relation to the solar wind magnetic field and magnetospheric substorms, J. Geophys. Res., 76, 4381-4401, 1971.
- Axford, W. Ian
- The interaction between the solar wind and the Earth's magnetosphere, J. Geophys. Res., 67, 3791-3796, 1962.
- Axford, W. Ian
- Viscous interaction between the solar wind and the Earth's magnetosphere, Planet. Space Sci., 12, 45-53, 1964.
- Axford, W. Ian
- The good old days, J. Geophys. Res., 99, 19,199-212, 1994.
- Axford, W.Ian and Colin O Hines
- A unifying theory of high-latitude geophysical phenomena and geomagnetic storms, Canad. J. Phys., 39, 1433-1464, 1961. Reprinted with comments in The Upper Atmosphere in Motion, C.O. Hines and Colleagues, p. 933-967, AGU, Washington, DC 1974.
- Axford, W. Ian, Harry E. Petschek and George L. Siscoe
- Tail of the magnetosphere, J. Geophys. Res., 70, 1231-1236, 1965.
- Bagenal, Fran
- Giant Planet Magnetospheres, Ann. Rev. Earth. Sci., 20, 289-328, 1992.
- Baker, Daniel N., E.W. Hones, Jr., P.R. Higbie, R.D. Belian and P. Stauning
- Global properties of the magnetosphere during a substorm growth phase: A case study, J. Geophys. Res., 86, 8941-8956, 1981.
- Barbier, Daniel
- L'activitŽ aurorale aux basses latitudes, Ann. Geophys., 14, 334-355, 1958.
- Birkeland, Kristian
- The Norwegian Aurora Polaris Expedition 1902-3, A. Aschehoug, Christiania, Norway, 1908, 1913.
- Bishop, Amasa S.
- Project Sherwood--The US Program of Controlled Fusion, 216 pp., Addison-Wesley, 1958.
- Blake, J. Bernard, W.A. Kolasinski, R.W. Fillius and E.G. Mullen
- Injection of electrons and protons with energies of tens of MeV into L<3 on 24 March 1991, Geophys. Res. Lett., 19, 821-824, 1992.
- Block, Lars P.
- Potential double layers in the ionosphere, Cosm. Electrodyn., 3, 349-376, 1972.
- Block, Lars P.
- A double layer review, Astrophys. Space Sci., 55, 59-83, 1978.
- Bostick, Winston H.
- Experimental study of ionized matter projected across a magnetic field, Phys. Rev., 104, 292-299, 1956.
- Bostick, Winston, H.
- Experimental study of plasmoids, Phys. Rev., 106, 404-412, 1957.
- Bostick, Winston H.
- What laboratory-produced plasma structures can contribute to the understanding of cosmic structures both large and small, IEEE Trans. on Plasma Sci., PS-14, 703-717, 1986.
- Bostrom, Carl O. and George H. Ludwig
- Instrumentation for Space Physics, Physics Today, 19, no.7, 43-56, July 1966.
- Bostrom, Rolf, A model of the auroral electrojets, J. Geophys Res., 69, 4983-4999, 1964.
- Bostrom, Rolf
- Currents in the ionosphere and magnetosphere, p. 445-458 in The Birkeland Symposium on Aurora and Magnetic Storms, A. Egeland and J. Holtet, editors, Centre de la Recherche Scientifique, Paris 1968.
- Boyd, John S.
- Rocket-borne measurements of auroral electrons, Rev. Geophys., 13, 735-740, 1975.
- Brice, Neil M.
- Bulk motion in the magnetosphere, J. Geophys. Res., 72, 5193-5211, 1967.
- Brice, Neil M.
- Differential Drift of Plasma Clouds in the Magnetosphere, unpublished manuscript, August 1973.
- Brown, W.L., W.N. Hess and J.A. Van Allen
- Introduction [to "Collected papers on the artificial radiation belt from the July 9, 1962, nuclear detonation], (c) J. Geophys Res., 68, 605-6, 1963.
- Brush, Stephen G.
- Prediction and theory evaluation: AlfvŽn on space plasma phenomena, Eos, 71, 19-33, January 9, 1990.
- Burch, James L., S.A. Fields and R.A. Heelis
- Polar cap electron acceleration regions, J. Geophys. Res., 84, 5863-5874, 1979.
- Burke, William J. M.C. Kelley, R.C. Sagalyn, M. Smiddy and S.T. Lai
- Polar cap electric field structure with northward interplanetary magnetic field, Geophys. Res. Let., 6, 21-24, 1979.
- Burton, Rande K., R.L. McPherron and C.T. Russell
- An empirical relationship between interplanetary conditions and Dst, J. Geophys. Res., 80, 4204-4214, 1975.
- Bythrow, Peter F. , Thomas A. Potemra and Lawrence J. Zanetti
- Variation of the auroral Birkeland current pattern associated with the north-south component of the IMF, p. 131-136 in Magnetospheric Currents, T.A. Potemra, ed., Amer. Geophys. Union, Washington, DC 1984.
- Caan, Michael N, Robert L. McPherron and Christopher T. Russell
- Substorm and interplanetary magnetic field effects on the geomagnetic tail lobes, J. Geophys. Res., 80, 191-194, 1975.
- Cahill, Lawrence J., Jr.
- Early study of the boundary of the geomagnetic field, in Physics of the Magnetopause, B.U.O.Sonnerup and P. Song, editors, p. 9-16, Amer. Geophys. Union, Washington, D.C. 1995.
- Cahill, Lawrence J., Jr. and P.G. Amazeen
- The boundary of the geomagnetic field, J. Geophys. Res., 68, 1835-1843, 1963.
- Carovillano, Robert L. and George L. Siscoe
- Energy and momentum theorems in magnetospheric physics, Rev. Geophys., 11, 289-353, 1973.
- Carpenter, Donald L.
- Whistler evidence of a "knee" in the magnetospheric ionization density profile, J. Geophys. Res., 68, 1675-1682, 1963.
- Carpenter, Donald L. and C.G. Park
- What ionospheric workers should know about the plasmapause-plasmasphere, Rev. Geophys., 11, 133-154, 1973.
- Carruthers, George R., T. Page and R.R. Meier
- Apollo 16 Lyman-alpha imagery of the hydrogen corona, J. Geophys. Res., 81, 1664-1672, 1976.
- Cauffman, David P. and Donald A. Gurnett
- Double-probe measurement of convection electric fields with the Injun 5 satellite, J. Geophys. Res., 76, 6014-6027, 1971.
- Cauffman, David P. and Donald A. Gurnett
- Satellite measurements of high latitude convection electric fields, Space Sci. Rev., 13, 369-410, 1972.
- Chapman, Sidney and Julian Bartels
- Geomagnetism, (2 vol.) 1049 pp.,Oxford Univ. Press, New York, 1940.
- Chree, C.
- Studies in Terrestrial Magnetism, 206 pp., McMillan & Co., London and New York, 1912 (also in Encyclopaedia Britannica, 11th ed. 1911, vol 17, pp. 353-385).
- Chiu, Yam T. and M. Schulz
- Self-consistent particle and parallel electrostatic field distributions in the magnetospheric-ionospheric auroral region, J. Geophys. Res., 83, 629-642, 1978.
- Christofilos, Nicholas C. (c)
- The Argus Experiment, J. Geophys. Res., 64, 869-876, 1959.
- Clauer, C.Robert and Robert L. McPherron
- Mapping the local time-universal time development of magnetospheric substorms using mid-latitude magnetic observations, J. Geophys. Res., 79, 2811-2820, 1974a.
- Clauer, C.Robert and Robert L. McPherron
- Variability of mid-latitude magnetic parameters used to characterize magnetospheric substorms, J. Geophys. Res., 79, 2898-2900, 1974b.
- Clauer, C. Robert, R.L. McPherron, C. Searls and M.G. Kivelson
- Solar wind control of auroral zone geomagnetic activity, Geophys. Res. lett., 8, 915-918, 1981.
- Cooper, John F. and J.A. Simpson
- Sources of high-energy protons in Saturn's magnetosphere, J. Geophys. Res., 85, 5793-5802, 1980.
- Corliss, William R.
- Scientific Satellites, 822 pp., NASA publication SP-133, U.S. Govt. Printing Office, 1967.
- Cowling, Thomas Gilbert
- On AlfvŽn's theory of magnetic storms and of the aurora, Terrestr. Magnetism and Atmosph. Electricity, 47, 209-214, 1942.
- Cowling, Thomas Gilbert
- Conductivity of an ionised gas, with applications, Proc. Roy. Soc. A., 183, 453-479, 1945.
- Coroniti, Frederick V. and Charles F. Kennel
- Changes in magnetospheric configuration during substorm growth phase, J. Geophys. Res., 77, 3361- 3370, 1972.
- Cummings, W.D. and A.J. Dessler
- Field-aligned currents in the magnetosphere, J. Geophys. Res., 72, 1007-1013, 1967.
- Dalgarno, Alexander, W.B. Hanson, N.W. Spencer and E.R. Schmerling (c)
- The Atmosphere Explorer mission, Radio Science, 8, 263-266, 1973.
- Dassoulas, John (c)
- The Triad spacecraft, APL Technical Digest (Applied Physics Laboratory of the Johns Hopkins Univ.), 12, 2-13, 1973.
- Davis, Leo R. and J. M. Williamson
- Low energy trapped protons, Space Research, 3, 365-375, North Holland Publ. Co., 1963.
- Davis, Leo R. and J.M. Williamson
- Outer zone protons, in Radiation Trapped in the Earth's Magnetic Field, Billy M. McCormac, editor, pp. 110-125, D. Reidel 1966.
- Davis, T. Neil
- The morphology of the auroral displays of 1957-1958, 2. Detail analyses of Alaska data and analyses of high-latitude data, J. Geophys. Res., 67, 75-110, 1962.
- Davis, T. Neil
- Magnetospheric convection pattern inferred from magnetic disturbance and auroral motion, J. Geophys. Res., 76, 5978-5984, 1971
- DeForest, Sherman E. and Carl E. McIlwain
- Plasma clouds in the magnetosphere, J. Geophys. Res., 76, 3587-3611, 1971.
- Dessler, Alex J.
- Discussion of paper by R.L. Arnoldy, R.A. Hoffman and J.R. Winckler, "Observation of the Van Allen radiation regions during August and September 1959," part 1, J. Geophys. Res., 65, 3487-3490, 1960.
- Dessler, Alex J. (editor)
- Physics of the Jovian Magnetosphere, xv + 544 pp., Cambridge Univ. Press, 1983.
- Dessler, Alex J.
- The Vernov radiation belt (almost), Science, 226, 915, 23 November 1984.
- Dessler, Alex J. and Eugene N. Parker
- Hydromagnetic theory of geomagnetic storms, J. Geophys. Res., 64, 2239-2252, 1959.
- Dessler, AlexJ. and Ernest H. Vestine
- Maximum total energy of the Can Allen Radiation Belt, J. Geophys. Res., 65, 1069-71, 1960.
- Dessler, Alex J. and Jules A.Fejer
- Interpretation of Kp index and M-region geomagnetic storms, Planet. Space Sci., 11, 505-511, 1963.
- Dessler, Alex J. and R.D. Juday
- Configuration of auroral radiation in space, Planet. Space Sci., 13, 63-72, 1965.
- DeVorkin, David H.
- Science with a Vengeance, xxii+404 pp., Springer-Verlag, 1992.
- Dobrowolny, M. and V. Formisano
- The structure of the Earth's bow shock, Rivista del Nuovo Cimento, 3, 419-489, 1973.
- Drake, Frank D.
- Discovery of the Jupiter Radiation Belts, in Serendipitous Discoveries in Radio Astronomy, K. Kellermann and B. Sheets, editors, p. 258-265, National Radio Astronomy Observatory, Green Bank, 1985.
- Dungey, J.W.
- Conditions for the occurence of electrical discharges in astrophysical systems, Philos. Mag., 44, 725-738 1953.
- Dungey, James W.
- Cosmical Electrodynamics, 183 pp., Cambridge Univ. Press, London 1958.
- Dungey, James W.
- Interplanetary magnetic field and the auroral zones, Phys. Rev. Letters, 6, 47-48, 1961.
- Dungey, James W.
- The structure of the exosphere, or, adventures in velocity in Geophysics, The Earth's Environment (Proceedings of the 1962 Les Houches Summer School), pp. 503-550, C. DeWitt, J. Hieblot and A. Lebeau, edditors, Gordon and Breach, NY 1963.
- Dungey, James W.
- The history of the magnetopause region, J.A.T.P., 40, 231-234, 1978.
- Dungey, James W.
- Memories, Maxims and Motives, J. Geophys. Res., 99, 19,189-197, 1994.
- Dungey, James W.
- Origins of the concept of reconnection and its application to the magnetopause: A historical view, in Physics of the Magnetopause, B.U.O.Sonnerup and P. Song, editors, p. 17-19, Amer. Geophys. Union, Washington, D.C. 1995a.
- Eather, Robert H.
- Majestic Lights, 323 pp., Amer. Geophys. Union, Washington, DC 1980.
- Elphic, Richard C.
- Fact, inference and speculation: what we know vs. what we believe about flux transfer events, in Physics of the Magnetopause, B.U.O.Sonnerup and P. Song, editors, Amer. Geophys. Union, Washington, D.C. (in press, 1994).
- Erickson, Gary M.
- On the cause of X-line formation in the near-earth plasma sheet: Results of adiabatic convection of plasma sheet plasma, p. 296-302 in Magnetic Reconnection in Space and Laboratory Plasmas, E.W. Hones, Jr., editor, AGU, Washington, DC, 1984.
- Erickson, Gary M.
- A quasi-static magnetospheric convection model in two dimensions, J. Geophys. Res., 97, 6505-6522, 1992.
- Erickson, Gary M. and Richard A. Wolf
- Is steady convection possible in the Earth's magnetotail? Geophys. Res. Let., 7, 897-900, 1980.
- Evans, David S.
- Precipitating electron fluxes formed by a magnetic field aligned potential difference, J. Geophys. Res., 79, 2853-58, 1974.
- Evans, David S.
- Evidence for low-altitude acceleration of auroral particles, p. 319-340 in Physics of Hot Plasma in the Magnetosphere, B. Hultqvist and L. Stenflo, eds., Plenum, NY, 1976a.
- Evans, David S.
- The acceleration of charged particles at low altitudes, p. 730-739 in Physics of Solar Planetary Environments, D.J. Williams, ed., Amer. Geophys. Union, Washington, DC 1976b.
- Ezell, Linda Neuman
- NASA Historical Data Book, Vol. II, Programs and Projects 1958-1968, 643 pp., NASA Publication SP-4012, 1988.
- Fahleson, Ulf
- Theory of electric field measurements conducted in the magnetosphere with electric probes, Space Sci. Rev., 7, 238-262, 1967.
- Fairfield, Donald H. and Lawrence G. Cahill, Jr.
- Transition region magnetic field and polar magnetic disturbances, J. Geophys. Res., 71, 155-169, 1966.
- Fairfield, Donald H.
- Polar magnetic disturbances and the interplanetary magnetic field, Space Research VIII, 107-119, 1967.
- Fairfield, Donald H. and Norman F. Ness
- Configuration of the geomagnetic tail during substorms, J. Geophys. Res., 75, 7032-7047, 1970.
- Farley, T.A.
- The growth of our knowledge of the Earth's outer radiation belt, Rev. Geophys., 1. 3-34, 1963.
- Feldshtein, Yakov I.
- Some problems concerning the morphology of auroras and magnetic disturbances at high latitude, Geomagnetism and Aeronomy, (English translation) 3, 183-192, 1963.
- Felds(h)tein, Yakov I.
- Polar auroras, polar substorms and their relationships with the dynamics of the magnetosphere, Rev. Geophys., 7, 179-218, 1969.
- Ferraro, Vincent C.A.
- Non-uniform rotation of the Sun and its magnetic field, Mon. Not. Roy.Astron.Soc., 97, 458-472, 1937.
- Forbes, Terry G. and Eric R. Priest
- A comparison of analytical and numerical models for steadily driven magnetic reconnection, Rev. Geophys., 25, 1583-1607, 1987.
- Forbush, Scott E.
- Three unusual cosmic-ray increases possibly due to charged particles from the sun, Phys. Rev., 70, 771-772, 1946.
- Foster, John S, T. Kenneth Fowler and Frederick E. Mills
- Nicholas C. Christofilos (obituary), Physics Today, 26, 109-115, January 1973.
- Frank, Louis A.
- Efficiency of a Geiger-Mueller tube for non-penetrating electrons, J. Franklin Inst., 273, 91-106, 1962.
- Frank, Louis A.
- On the extraterrestrial ring current during geomagnetic storms, J. Geophys. Res., 72, 3753-67, 1967 (ring current ions with OGO 3).
- Frank, Louis A.
- Relationship of the plasma sheet, ring current trapping boundary and plasmapause near the magnetic equator and local midnight, J. Geophys. Res., 76, 6971-6979, 1971.
- Frank, Louis A., et al.
- The theta aurora, J. Geophys. Res., 91, 3177-3224, 1986.
- Frank, Louis A. and Kenneth L. Ackerson
- Observations of charged particle precipitation into the auroral zone, J. Geophys. Res., 76, 3612-3643, 1971.
- Frank, Louis A., K.L. Ackerson and R.P. Lepping
- On hot tenuous plasmas, fireballs and boundary layers in the earth's magnetotail, J. Geophys. Res., 81, 5859-5881, 1976.
- Frank, Louis A. and K.L. Ackerson
- Reply, J. Geophys. Res., 82, 5641-5643, 1977.
- Frank, Louis A., R.J. DeCoster and K.L. Ackerson
- Reply, J. Geophys. Res., 83, 1187-1190, 1978a.
- Frank, Louis A., R.J. DeCoster and K.L. Ackerson
- Reply, J. Geophys. Res., 83, 3365-3366, 1978b.
- Frank, Louis A. and Kenneth L. Ackerson
- Several recent findings concerning the dynamics of the Earth's magnetotail, Space Science Reviews, 23, 375-392, 1979.
- Frank, Louis A. and John D. Craven
- Imaging results from Dynamics Explorer 1, Rev. Geophys., 26, 249-283, 1988.
- Frank, Louis A. with Patrick Huyghe
- The Big Splash, 255 pp., Birch Lane press, Carol Publ. Group, New York, 1990.
- Franklin, Kenneth L.
- An account of the discovery of Jupiter as a radio source, Astron J., 64, 37-39, 1959.
- Franklin, Kenneth L.
- The discovery of Jupiter Bursts, in Serendipitous Discoveries in Radio Astronomy, K. Kellermann and B. Sheets, editors, p. 252-257 National Radio Astronomy Observatory, Green Bank, 1985.
- Freden, S.C. and R.S. White
- Protons in the Earth's magnetic field, Phys. Rev. Letters, 3, 9-11, 1959.
- Friedman, Herbert
- From ionosonde to rocket sonde, J. Geophys. Res., 99, 19,143-153, 1994.
- Friis-Christensen, Egil, K. Lassen, J.M. Wilcox, W. Gonzalez and D.S. Colburn
- Interplanetary magnetic sector polarity from polar geomagnetic field observations, Nature (Physical Sciences), 233, PS48-PS50, 20 September 1971.
- Fritz, Hermann
- Das Polarlicht, Brockhaus, Leipzig, 1881.
- Fujii, R., Takesi Iijima, Thomas A. Potemra and Masahisa Sugiura
- Seasonal dependence of large-scale Birkeland currents, Geophys. Res. Lett., 8, 1103-1106, 1981.
- Fukushima, Naoshi
- Equivalence in ground geomagnetic effect of Chapman-Vestine's and Birkeland-Alfven's current systems for polar magnetic storms, Rep. Ionos.Space Res.Jap., 23, 219-227, 1969.
- Fukushima, Naoshi
- Generalized theorem for no ground magnetic effect of vertical currents connected with Pedersen currents in the uniform-conductivity ionosphere, Rep. Ionos.Space Res.Jap., 30, 35-40, 1976.
- Fukushima, Naoshi
- Some topics and historical episodes in geomagnetism and aeronomy, J. Geophys. Res., 99, 19,113-142, 1994.
- Giovanelli, R.G.
- Magnetic and electric phenomena in the sun's atmosphere associated with sunspots, Month. Not. Roy. Astr. Soc., 107, 338-355, 1947.
- Goertz, Christoph K.
- Double layers and electrostatic shocks in space, Rev. Geophys., 17, 418-426, 1979.
- Goertz, Christoph K. and Robert A. Smith
- The thermal catastrophe model of substorms, J. Geophys. Res., 94, 6581-96, 1989.
- Gold, Thomas
- Motions in the magnetosphere of the Earth, J. Geophys. Res., 64, 1219-1224, 1959.
- Gombosi, Tamas I., Bengt Hultqvist and Yohsuke Kamide, editors (c)
- Pioneers of Space Physics, J. Geophys. Res., 99, 19,099-212, 1994.
- Gosling, John T.
- The solar flare myth, J. Geophys. Res., 98, 18,937-18,949, 1993.
- Grad, Harold
- Variational principle for a guiding center plasma, Phys. Fluids, 9, 225-251, 1966.
- Greenstadt, Eugene W. and Robert W. Fredricks
- Shock systems in collisionless space plasmas, p. 3-43 in vol 3 of Solar System Plasma Physics, C.F. Kennel, L.J. Lanzerotti and E.N. Parker, editors, North Holland 1979.
- Gurnett, Donald A.
- The earth as a radio source: terrestrial kilometric radiation, J. Geophys. Res., 79, 4227-4238, 1974.
- Gustafsson, Georg
- On the orientation of auroral arcs, Planet. Space Sci., 15, 277-294, 1967.
- Hallinan, Thomas J.
- Auroral spirals, 2. Theory, J. Geophys. Res., 81, 3959-3965, 1976.
- Hanson, William B. and R. A. Heelis
- Techniques of measuring bulk gas motions from Satellites, Space Sci. Instr., 1, 493-524, 1975.
- Hapgood, M.A.
- Space physics coordinate transformations: a user's guide, Planet. Space Sci., 40, 711-717, 1992.
- Harang, Leiv
- The Aurorae, x + 166 pp., John Wiley & Sons, New York, 1951.
- Hardy, David A.
- H.Kent Hills and John W. Freeman, Jr., A new plasma regime in the distant geomagnetic tail,Geophys. Res. Lett. , 2, 169-172, 1975
- Hardy, David A., H. Kent Hills and John W. Freeman, Jr.
- Occurence of lobe plasma at lunar distance, J. Geophys. Res., 84, 72, 1979.
- Hau, Lin-Ni, R.A. Wolf, C.H. Voigt and C.C. Wu
- Steady state magnetic field configurations for the Earth's magnetotail, J. Geophys. Res., 94, 1303-16, 1989.
- Heelis, Rodney A., W.B. Hanson and J.L. Burch
- Ion convection velocity reversals in the dayside cleaft, J. Geophys. Res., 81, 3803-3809, 1976.
- Heelis, Rodney A., W.B. Hanson, C.R. Lippincott, L.H. Harmon, B.J. Holt, J.E. Doherty and R.A. Power
- The ion drift meter for Dynamics Explorer B, Space Sci. Instrum., 5, 511-521, 1981.
- Helliwell, Robert A.
- Whistlers and Related Ionospheric Phenomena, 349 pp., Stanford University Press, Stanford, CA 1965.
- Heppner, James P.
- The world magnetic survey, Space Sci. Rev., 2, 315-354, 1963.
- Heppner, James D.
- Recent measurements of the magnetic field in the outer magnetosphere and boundary regions, Space Sci. Rev., 7, 166-190, 1967.
- Heppner, James P.
- Electric field variations during substorms: Ogo-6 measurements, Planet. Space Sci., 20, 1475-1498, 1972a.
- Heppner, James P.
- The Harang discontinuity in auroral belt ionospheric currents, Geophys. Publ., 29, 105-120, 1972b.
- Heppner, James P.
- Polar cap electric field distributions related to the interplanetary magnetic field direction, J. Geophys. Res., 77, 4877-4887, 1972c.
- Heppner, James P.
- Empirical models of high-latitude electric fields, J. Geophys. Res., 82, 1115-1125, 1977.
- Heppner, J.P., N.F. Ness, C.S. Scearce a,d T.L. Skillman
- Explorer 10 magnetic field measurements, J. Geophys. Res., 68, 1-46. 1963.
- Heppner, James P. and Nelson C. Maynard
- Empirical high-latitude electric field models, J. Geophys. Res., 92, 4467-4489, 1987.
- Hess, Wilmot N.
- Energetic perticles in the inner Van Allen Belt, Space Sci. Rev., 1, 278-312, 1962.
- Hess, Wilmot N., editor
- The Physics of Solar Flares (proc. of AAS-NASA Symposium), NASA SP-50, 1964.
- Hess, Wilmot N.
- The Radiation Belt and Magnetosphere, viii+548 pp., Blaisdell Publ. Co., Waltham 1968.
- Hess, Wilmot N., G. Mead and M.P. Nakada
- Advances in particles and field research in the satellite era, Reviews of Geophysics, 3, 521-570, 1965.
- Hesse, Michael and Karl Schindler
- A theoretical foundation of general magnetic reconnection, J. Geophys. Res., 93, 5559-5567, 1988.
- Hines, Colin
- The magnetopause: a new frontier in space, Science, 141, 130-136, 1963.
- Hines, Colin O.
- Hydromagnetic motions in the magnetosphere, Space Sci. Rev., 3, 342-379, 1964
- Hines, Colin O. and colleagues
- The Upper Atmosphere in Motion, 1027 pp., Geophysical Monograph 18, Amer. Geophys. Union, Washington, D.C. 1974.
- Hines, Colin O.
- Origins of the 1961 Axford-Hines paper on magnetospheric convection, Eos, 67, 634, 1986. (Reprinted in History of Geophysics, 4, S. Gillmor, editor, pp. 97-98, Amer. Geophys. Union, Washington, D.C. 1990)
- Hoffman, Robert A.
- The magnetosphere, ionosphere and atmosphere as a system: Dynamics Explorer 5 years later, Rev. Geophys., 26, 209-214, 1988.
- Hoffman, Robert A., G.D. Hogan and R.C. Maehl (c)
- Dynamics Explorer spacecraft and ground operations systems, Space Sci. Instrument., 5, 349-367, 1981.
- Holzer, Robert E. and J.A. Slavin
- Magnetic flux transfer associated with expansions and contractions of the dayside magnetosphere, J. Geophys. Res., 83, 3831-3839, 1978.
- Hones, Edward W., Jr.
- The magnetotail: its generation and dissipation, in Physics of Solar-Planetary Environments, pp. 558-571, D.J. Williams, editor, Amer. Geophys. Union, Washington, D.C., 1976.
- Hones, Edward J., Jr.
- Substorm processes in the magnetotail: Comments on 'On hot tenuous plasmas, fireballs, and boundary layers in the Earth's magnetotail' by L.A. Frank, K.L. Ackerson and R.P. Lepping, J. Geophys. Res., 82, 5633-40 , 1977.
- Hones, Edward W. Jr.
- Comment on "on hot tenuous plasmas, fireballs and boundary layers in the Earth's magnetotail" by L.A. Frank. K.L. Ackerson and R.P. Lepping, J. Geophys. Res., 83, 1183-1186, 1978a.
- Hones, E.W. Jr.
- Processes at the magnetotail boundary: Comment on "on hot tenuous plasmas, fireballs and boundary layers in the Earth's magnetotail" by L.A. Frank. K.L. Ackerson and R.P. Lepping, J. Geophys. Res., 83, 2216-2227, 1978b.
- Hones, E.W. Jr.
- Some characteristics of rapidly flowing magnetotail plasmas: further comments on "on hot tenuous plasmas, fireballs and boundary layers in the Earth's magnetotail" by L.A. Frank. K.L. Ackerson and R.P. Lepping, J. Geophys. Res., 83, 3358-3364, 1978c.
- Hones, Edward W., Jr.
- Transient phenomena in the magnetotail and their relation to substorms, Space Sci. Rev., 23, 393-410, 1979.
- Hones, Edward W., Jr. (ed.)
- Magnetic Reconnection in Space and Laboratory Plasmas, Geophysical Monograph 30, AGU, Washington, DC, 1984a.
- Hones, Edward W., Jr.
- Historical note on Ronald G. Giovanelli, pp. v-vi in, Magnetic Reconnection in Space and Laboratory Plasmas, AGU, Washington, DC, 1984b.
- Hones, Edward W., Jr.
- J.R. Asbridge, S.J. Bame and I.B. Strong, Outward flow of plasma in the magnetotail following geomagnetic bays, J. Geophys. Res., 72, 5879-92, 1967.
- Hones, Edward W., Jr.
- S.I. Akasofu, P. Perreault, S.J. Bame and Sidney Singer, Poleward expansion of the auroral oval and associated phenomena in the magnetotail during auroral substorms, J. Geophys. Res., 75, 7060-7074, 1970.
- Huang, Cheryl Y. and Louis A. Frank
- A statistical study of the central plasma sheet: Implications for substorm models, Geophys. Res. Let., 13, 652-655, 1986.
- Hughes, W.Jeffrey
- Hydromagnetic Waves in the Magnetosphere, Rev. Geophys., 21, 508-520, 1983.
- Hultqvist, Bengt (c)
- The Viking project. Geophys. Res. Lett, 14, 379-382, 1987.
- Hunten, Donald, M. and Thomas M. Donahue
- Hydrogen glows from the terrestrial planets, Annu. Rev. Earth. Planet. Sci., 4, p. 265-292, 1976.
- Iijima, Takesi and Thomas A. Potemra
- The amplitude distribution of field-aligned currents at northern high latitudes observed by Triad, J. Geophys. Res., 81, 2165-2174, 1976a.
- Iijima, Takesi and Thomas A. Potemra
- Field-aligned currents in the dayside cusp observed by Triad, J. Geophys. Res., 81, 5971-5979, 1976b.
- Ismail, Syed, D.D. Wallis and L.L. Cogger
- Characteristics of polar cap sun-aligned arcs, J. Geophys. Res., 82, 4741-4749, 1977.
- Iyemori, T., H. Maeda,and T. Kamei
- Impulse response of geomagnetic indices to interplanetary magnetic field, J. Geomag. Geoelectr.,31, 1-9, 1979.
- Jaggi, Radhe K. and Richard A. Wolf
- Self-consistent calculation of the motion of a sheet of ions in the magnetosphere, J. Geophys. Res., 78, 2852-2866, 1973.
- Jackson, John E. and James I. Vette
- OGO Program Summary, 310 pp.NASA document SP-7601, NASA, Washington, D.C. 1975.
- Johnson, Francis S.
- The gross character of the geomagnetic field in the solar wind, J. Geophys. Res., 65, 3049-3051, 1960.
- Johnson, Francis S.
- The driving force for magnetospheric convection, Rev. Geophys., 16, 161-167, 1978.
- Johnson, Richard G
- Energetic ion composition in the Earth's magnetosphere, Rev. Geophys., 17, 696-704, 1979.
- Kan. Joseph R.
- Developing a global model of magnetospheric substorms, Eos, 71, 1083, 1086-7, 18 September 1990.
- Kan, Joseph R., L. Zhu and S.-I. Akasofu
- A theory of substorms: onset and subsidence, J. Geophys. Res., 93, 5624-5640,1988.
- Keath, Edwin P, E.C. Roelof, C.O. Bostrom and D.J. Williams, Roelof
- Fluxes of >50 keV protons and >30 keV electrons at about 35 RE 2. Morphology and flow patterns in the magnetotail, J. Geophys. Res., 81, 2315-26, 1976.
- Kelley, Michael, C.
- The Earth's Ionosphere--Plasma Physics and Electrodynamics, ix + 487 pp., Academic Press, 1989.
- Kellogg, Paul J.
- Possible explanation of the radiation observed by Van Allen at high altitudes in satellites, Nuovo Cimento, 11, 48-66, 1959.
- Kellogg, Paul J.
- Flow of plasma around the Earth, J. Geophys. Res., 67, 3805-3811, 1962.
- Kennel, Charles F.
- Consequences of a Magnetospheric Plasma, Rev. Geophys., 7, 379-419, 1969.
- Kennel, Charles F.
- Neil Mather Brice, 1934-1974, Eos, 66, 1353-1355, 1985. (Reprinted in History of Geophysics, 4, S. Gillmor, editor, pp. 253-255, Amer. Geophys. Union, Washington, D.C. 1990.)
- Kennel, Charles F.
- Critical Mach numbers in classical magnetohydrodynamics, J. Geophys. Res., 92, 13,427-13,437, 1987.
- Kennel. Charles F., J.P. Edmiston and T. Hada
- A quarter century of collisionless shock research, in Collisionless shocks in the heliosphere: a tutorial review, R. G. Stone and B.T. Tsurutani, editors, pp. 1-36, Geophysical Monograph 34, Amer. Geophys. Union, Washington, D.C. 1985.
- Kennel, Charles F. and Harry E. Petschek
- Limit on stably trapped particle fluxed, J. Geophys. Res., 71, 1-28, 1966.
- Knight, S.
- Parallel electric fields, Planet, Space Sci., 21, 741-750, 1973.
- Langel, Robert A.
- Near-Earth magnetic disturbance in total field at high latitude, 1. Summary of data from Ogo 2, 4 and 6, J. Geophys. Res., 79, 2663--2671, 1974
- Lanzerotti, Louis J. and David J. Southwood
- Hydromagnetic waves, p. 109-135 in vol. 3 of Solar System Plasma Physics, C.F. Kennel, L.J. Lanzerotti and E.N. Parker, eds., North Holland 1979.
- Lee, Lou C. and Z.F. Fu
- A Theory of magnetic flux transfer at the Earth's magnetopause, Geophys. Res. Lett.,12, 105-108, 1985.
- Le Galley, Donald P.
- Space Science, xviii+668 pp., John Wiley and Sons, 1963.
- Lemaire, Joseph
- The mechanisms of formation of the plasmapause, Ann. Geophys., 31, 175-190, 1975.
- Lepping, Ronald P.
- Characteristics of the magnetopauses of the magnetized planets, in Physics of the Magnetopause, B.U.O.Sonnerup and P. Song, editors, p. 61-70, Amer. Geophys. Union, Washington, D.C. 1995.
- Levy, R.H., H.E. Petschek and G.L. Siscoe
- Aerodynamic aspects of the magnetospheric flow, AIAA J., 2, 2065-2076, 1964.
- Liemohn, Harold B.
- The lifetimes of radiation belt protons with energies between 1 keV and 1 Mev, J. Geophys. Res., 66, 3593-3595, 1961.
- Longanecker, Gerald W., and R.A. Hoffman (c)
- S3-A spacecraft and experiment description, J. Geophys. Res., 78, 4711-4717, 1973.
- Lopez, Ramon E. and Daniel N.Baker
- Evidence for particle acceleration during magnetospheric substorms, Astroph.J. Suppl., 90, 531-539, 1994.
- Ludwig, George H.
- The orbiting geophysical observatories, Space Sci. Rev., 2, 175-218, 1963.
- Lui, Anthony T.Y.
- Extended consideration of a synthesis model for magnetospheric substorms, in Magnetospheric Substorms, J.R. Kan, T.A. Potemra, S. Kokubun and T. Iijima, editors, p. 43-60, Geophysical Monograph 64, Amer. Geophys. Union, Washington, D.C. 1991
- Lui, Anthony T.Y. and C.D. Anger
- A uniform belt of diffuse auroral emission seen by the Isis-2 scanning photometers, Planet. Space Sci., 21, 799-809, 1973.
- Lui, Anthony T.Y.
- R.W. McEntire and S.M. Krimigis, Evolution of the ring current during two geomagnetic storms, J. Geophys. Res., 92, 7459-7470, 1987.
- Lui, Anthony T.Y. and D.C. Hamilton
- Radial profiles of quiet-time magnetospheric parameters, J. Geophys. Res., 97, 19,325--19,332, 1992.
- Manka, Robert H., T.A. Fritz, R.G. Johnson, R.A. Wolf, M.J. Teague and J.I. Vette
- Overview of the IMS July 29, 1977, Magnetic Storm Analysis, J. Geophys. Res., 87, 5871-5880, 1982.
- Mansurov, S.M.
- New evidence of a relationship between magnetic fields in space and on Earth, Geomag. Aeron., 9, 622-623, 1969.
- Mauk, Barry H. and C.-I. Meng
- Plasma injection during substorms, Physica Scripta, T18, 128-139, 1987.
- Mayaud, P.N.
- Derivation, Meaning and Use of Geomagnetic Indices,
vi+154 pp., Geophysical Monograph 22, Amer. Geophys. Union, 1980.
- McIlwain, Carl E.
- Direct measurement of particles producing visible auroras, J. Geophys. Res., 65, 2727-2747, 1960.
- McIlwain, Carl E.
- The radiation belts, natural and artificial, Science, 142, 355-361, 1963.
- McPherron, Robert L.
- Growth phase of magnetospheric substorms, J. Geophys. Res., 75, 5592-5599, 1970.
- McPherron, Robert L.
- Substorm related changes in the geomagnetic tail: the growth phase, Planet. Space Sci., 20, 1521-39, 1972.
- McPherron, Robert L.
- Magnetospheric Substorms, Rev. Geophys., 17, 657-681, 1979.
- McPherron, Robert L., C. T. Russell and M. P. Aubry
- Satellite studies of magnetospheric substorms on August 15, 1968, 9., Phenomenological model for substorms, J. Geophys. Res., 78, 3131-3149, 1973.
- McPherron, Robert L. and J.N. Barfield
- A seasonal change in the effect of field-aligned currents at synchronous orbit, J. Geophys. Res., 85, 6743-6746, 1980.
- Mencke-Hansen, A., A. Bahnsen and N. D'Angelo
- The cusp-magnetosheath interface, J. Geophys. Res., 81, 556-561, 1976.
- Meng, C.-I., R.H. Holzworth and S.-I. Akasofu
- Auroral circle--delineating the poleward boundary of the quiet auroral belt, J. Geophys. Res., 82, 164-172, 1977.
- Meredith, Leslie H., M.B. Gottlieb and J.A. Van Allen
- Direct detection of soft radiation above 50 kilometers in the auroral zone, Phys. Rev., 97, 201-205, 1955.
- Mizera, Paul F. et al.
- The aurora inferred from S3-3 particles and fields, J. Geophys. Res., 86, 2329-2339, 1981.
- Mozer, Forrest et al.
- Observation of paired electrostatic shocks in the polar magnetosphere, Phys. Rev. Lett., 38, 292-295, 1977.
- Nagai, Tsugunobu, D.N. Baker and P.R. Higbie
- Development of substorm activity in multiple-onset substorms at synchronous orbit, J. Geophys. Res., 88, 6994-7004, 1983.
- Naugle, John E. (introducer)
- Scientific Findings from Explorer VI, 381 pp., NASA document SP-54, U.S. Printing Office, 1965.
- Ness, Norman F.
- The Earth's magnetic tail, J. Geophys. Res., 70, 2989-3005, 1965.
- Ness, Norman F.
- The geomagnetic tail, Rev. Geophys., 7, 97-127, 1969.
- Ness, Norman F.
- Magnetometers for space research, Space Sci. Rev., 11, 459-554, 1970.
- Ness, Norman F.
- The magnetic fields of Mercury, Mars and Moon, Ann. Rev. Earth Planet. Sci., 7, 249-288, 1979.
- Ness, Norman F.
- Magnetotail research: the early years, pp. 11-20 in Magnetotail Physics, edited by A.T.Y. Lui, The Johns Hopkins University Press, 1987.
- Ness, Norman F., C.S.Scearce and J.B. Seek
- Initial results of the IMP-1 magnetic field experiment, J. Geophys. Res., 69, 3531-3569, 1964.
- Ness, Norman F., M.H. Acuna, R.P. Lepping, L.F.Burlaga, K.W.Behannon and F.M.Neubauer
- Magnetic field studies at Jupiter by Voyager 2: Preliminary results, Science, 206, 966-972, 1979.
- Newcomb, William A.
- Motion of magnetic lines of force, Ann. Phys., NY, 3, 347-385, 1958.
- Newell, Homer E., Jr., (editor)
- Sounding Rockets, 334 pp., McGraw Hill, NY 1959.
- Newell, Homer E., Jr.
- Beyond the Atmosphere, NASA Special Publication 4211, xviii + 497 pp., , Govt. Printing Office, Washington, DC 1980.
- Nishida, Atsuhiro
- Formation of plasmapause, or magnetospheric plasma knee, by the combined action of magnetospheric convection and plasma escape from the tail, J. Geophys. Res., 71, 5669-5679, 1966.
- Nishida, Atsuhiro, and T. Mukai, T. Yamamoto, Y. Saito, S. Kokubun and K. Maezawa
- Geotail observations of magnetospheric convection in the distant tail at 200 RE in quiet times, J. Geophys. Res., 100, 22,663-22,675 , 1995.
- Northrop, T.G. and E. Teller
- Stability of the adiabatic motion of charged particles in the earth's field, Phys. Rev., 117, 215-225, 1960.
- O'Brien, Brian J.
- Lifetime of outer zone electrons and their precipitation into the atmosphere, J. Geophys. Res., 67, 3687-3706, 1962.
- O'Brien, Brian J.
- Review of studies of trapped radiation with satellite-borne apparatus, Space Science Reviews, 1, 415-484, 1963.
- O'Brien, Brian J.
- Energetic charged particles in the magnetosphere, p. 169-211 in Solar-Terrestrial Physics, J.W. King and W.S. Newman, eds., Academic Press, London 1967.
- O'Brien, Brian J., C.D. Laughlin and D.A. Gurnett (c)
- High-latitude geophysical studies with satellite Injun 3, 1. Description of the satellite, J. Geophys. Res., 69, 1-12, 1964.
- O'Brien, Brian J. and H. Taylor
- High-latitude geophysical studies with the satellite Injun III, 4. Auroras and their excitation, J. Geophys. Res., 69, 45-63, 1964.
- Odishaw, Hugh and Stanley Ruttenberg (eds.)
- Geophysics and the IGY, v+210 pp., Geophysical Monograph 2, American Geophysical Union, 1958.
- Ogilvie, Keith W., A. Durney and T. von Rosenvinge (c)
- Description of experimental investigations and instruments for the ISEE spacecraft, IEEE Trans. Geoscience Electr., GE-16, 151-153, 1978.
- Parker, Eugene N.
- The solar-flare phenomenon and the theory of reconnection and annihilation of magnetic fields, Astrophys. J. Suppl., 8, Supplement #77, 177-211, 1963.
- Papadopoulos, Konstantinos
- A review of anomalous resistivity for the ionosphere, Rev. Geophys., 15, 113-127, 1977.
- Paschmann, Goetz, G. Haerendel, N. Sckopke and H. Rosenbauer
- Plasma and magnetic field characteristics of the distant polar cusp near local noon: the entry layer, J. Geophys. Res., 81, 2883-2899, 1976.
- Paschmann, Goetz, B.U.O. Sonnerup, I. Papamastorakis, N. Sckopke, G. Haerendel, S.J.
- Bame, J.R. Asbridge, J.T. Gosling, C.T. Russell and R.C. Elphic
Plasma acceleration at the Earth's magnetopause: evidence for reconnection, Nature, 282, 283-286, 1979.
- Perreault, Paul and Syun-Ichi Akasofu
- A study of geomagnetic storms, Geophys. J. Roy. Astr. Soc., 54, 547-573, 1978.
- Persson, Hans
- Electric field along a magnetic line of force in a low-density plasma, Phys. Fluids, 6, 1756-1759, 1963.
- Persson, Hans
- Electric field parallel to the magnetic field in a low density plasma, Phys. Fluids, 9, 1090-1098, 1966.
- Petschek, Harry E.
- Magnetic field annihilation, p. 425-439 in The Physics of Solar Flares (proc. of AAS-NASA Symposium), Wilmot N. Hess, ed., NASA SP-50, 1964.
- Petschek, Harry E.
- Concept of fast reconnection, in Physics of the Magnetopause, B.U.O.Sonnerup and P. Song, editors, p. 21-28Amer. Geophys. Union, Washington, D.C., 1995.
- Pickering, William H.
- History of the Juno cluster system, in Astronautical Engineering and Science, pp. 201-214., Ernest Stuhlinger, Frederick I. Ordway III, Jerry C. McCall and George C. Bucher, editors, McGraw Hill, 1963.
- Pike, Charles P. and J.A. Whalen
- Satellite observations of auroral substorms, J. Geophys. Res., 79, 985-1000, 1974.
- Pomerantz, Martin A.
- Scott E. Forbush 1904-1984, Eos, 65, 473-474, 1984 (reprinted in p. 187-189 of History of Geophysics, vol 2, C.S. Gillmor, ed., Amer. Geophys. Union, Washington, DC 1986).
- Rees, Manfred H. and R.G. Roble
- Observations and theory of the formation of stable auroral arcs, Rev. Geophys., 13, 201-242, 1975.
- Reiff, Patricia H., Robert W. Spiro and Thomas W. Hill
- Dependence of polar cap potential drop on interplanetary parameters, J. Geophys. Res., 86, 7639-7648, 1981 (erratum, 87, 2579).
- Reiff, Patricia and J.L. Burch
- IMF By-dependent plasma flow and Birkeland currents in the dayside magnetosphere, 2. A global model for northward and southward IMF, J. Geophys. Res., 90, 1595-1609, 1985.
- Rich, Frederick J. and M. Susan Gussenhoven
- The absence of region 1/region 2 field-aligned currents during prolonged quiet times, Geophys. Res. Lett., 14, 689-692, 1987.
- Roelof, Edward C., E.P. Keath and T. Iijima
- Fluxes of >50 keV protons and >30 keV electrons at about 35 RE 1. Velocity anisotropies and plasma flow in the magnetotail, J. Geophys. Res., 81, 2304-14, 1976.
- Roelof, Edward C. and David G. Sibeck
- Magnetopause shape as bivariate function of interplanetary magnetic field Bz and solar wind dynamic pressure, J. Geophys. Res., 98, 21,421-450, 1993; correction, J. Geophys. Res., 99, 8787-88, 1994.. [2.6]
- Rosenbauer, Helmut, H. GrŸnwaldt, M.D. Montgomery, G. Paschmann and N. Sckopke
- Heos 2 plasma observations in the distant polar magnetosphere: the plasma mantle, J. Geophys. Res., 80, 2723-2737, 1975.
- Rosenbluth, Marshall N. and Conrad L. Longmire
- Stability of plasma confined by magnetic fields, Ann. Phys., 1, 120-140, 1957.
- Rostoker, Gordon
- Polar magnetic substorms, Rev. Geophys., 10,157-211, 1972.
- Rostoker, Gordon
- Geomagnetic indices, Rev. Geophysics, 10, 935-950, 1972.
- Rostoker, Gordon, S.-I. Akasofu, J. Foster, R.A. Greenwald, Y. Kamide, K. Kawasaki, A.T.Y. Lui, R.L. McPherron and C.T. Russell
- Magnetospheric Substorms--definitions and signatures, J. Geophys. Res., 85, 1663-1668, 1980.
- Rostoker, Gordon and Timothy Eastman
- A boundary layer model for magnetospheric substorms, J. Geophys. Res., 92, 12,187-12,201, 1987.
- Rufenach, Clifford L., R.L. McPherron and J. Schaper
- The quiet geomagnetic field at geosynchronous orbit and its dependence on solar wind dynamic pressure, J. Geophys. Res., 97, 25-42, 1992.
- Russell, Christopher T.
- Geophysical coordinate transformations, Cosmic Electrodyn., 2, 184-196, 1971.
- Russell, Christopher T. and Richard C. Elphic
- Initial ISEE magnetometer results: magnetopause observations, Space Sci. Rev., 22, 681-715, 1978.
- Russell, Christopher T. and Richard C. Elphic
- ISEE observations of flux transfer events at the dayside magnetopause, Geophys. Res. Let., 6, 33-36, 1979.
- Schield, Milo A., J. W. Freeman and A. J. Dessler
- A source for field-aligned currents at auroral latitudes, J. Geophys. Res., 74, 247-256, 1969.
- Schindler, Karl and J. Birn
- Magnetospheric physics, Physics Reports, 47, 109-165, 1978.
- Science (Pioneer 10 at Jupiter) 183, 301-324, 1974.
- Science (Mariner 10 at Mercury), 185, 141-184, 1975a.
- Science (Pioneer 11 at Jupiter), 188, 445-477, 1975.
- Science (Voyager 1 at Jupiter), 204, 945-1008, 1979a.
- Science (Voyager 2 at Jupiter), 206, 925-996, 1979b.
- Science (Pioneer 11 at Saturn), 207, 400-453, 1980.
- Science (Voyager 1 at Saturn), 212, 159-243, 1981.
- Science (Voyager 2 at Saturn), 215, 499-594, 1982.
- Science (Voyager 2 at Uranus), 233, 39-109, 1986.
- Science (Voyager 2 at Neptune), 246, 1417-1501, 1989.
- Science (Ulysses at Jupiter), 256, 1487-1502, 1992.
- Sckopke, Norbert
- A general relation between the energy of trapped particles and the disturbance field near Earth, J. Geophys. Res., 71, 3125-3130, 1966.
- Sharp, Richard D., Richard G. Johnson, Edward G. Shelley and K.K. Harris
- Energetic O+ ions in the magnetosphere, J. Geophys. Res., 79, 1844-1850, 1974.
- Sharp, Richard D., R.G. Johnson and E.G. Shelley
- Observations of an ionosphric acceleration mechanism producing energetic (keV) ions primarily normal to the geomagnetic field directions, J. Geophys. Res., 82, 3324-3328, 1977.
- Shawhan, Stanley D.
- Magnetospheric plasma waves, p. 211-270 in vol. 3 of Solar System Plasma Physics, C.F. Kennel, L.J. Lanzerotti and E.N. Parker, eds., North Holland 1979.
- Shelley, Edward G., R.G. Johnson and R.D. Sharp
- Satellite observations of energetic heavy ions during a geomagnetic storm, J. Geophys. Res., 77, 6104-6110, 1972.
- Shelley, Edward G., R.D. Sharp and R.G. Johnson
- Satellite observations of an ionospheric acceleration mechanism, Geophys. Res. Lett., 3, 654-656, 1976.
- Shepherd, Gordon G.
- Dayside cleft aurora and its ionospheric effects, Rev. Geophys., 17, 2017-2033, 1979.
- Sibeck, David G., R.E. Lopez and E.C. Roelof
- Solar wind control of the magnetopause shape, location and motion, J. Geophys. Res., 96, 5489-5495, 1991.
- Silsbee, H.C. and E.H. Vestine
- Geomagnetic bays, their frequency and current systems, J. Geophys. Res., 47, 195-208, 1942.
- Simpson, J.A.
- A physicist in the world of geophysics and space, J. Geophys. Res., 99, 19,159-73, 1995.
- Singer, S. Fred
- A new model of magnetic storms and aurorae, Eos (Transact. Amer. Geophys. Union), 38, 175-190, 1957
- Singer, S. Fred
- "Radiation belt" and trapped cosmic ray albedo, Phys.Rev. Letters, 1, 171-173, 1958.
- Singer, S.Fred and Allen M. Lencheck
- Geomagnetically trapped radiation, Progress in Elementary Particle and Cosmic Ray Physics, VI, p. 247-335, North Holland Publ., Amsterdam 1962.
- Siscoe, George L.
- What's in the name "Magnetospheric substorm"?, J. Geophys. Res., 85, 1643-4, 1980.
- Slavin, James A., Edward J. Smith, David G. Sibeck, Daniel N. Baker, Ronald D. Zwickl and Syun-Ichi Akasofu
- An ISEE 3 study of the average and substorm conditions in the distant magnetotail, J. Geophys. Res., 90, 10,875-10,895, 1985.
- Smith, Edward J.
- Theoretical and experimental aspects of ring currents, in Space Science, edited by Donald LeGalley, pp. 316-373, John Wiley, New York, 1963.
- Smith, Paul H., Robert A. Hoffman and Theodore A. Fritz
- Ring current proton decay by charge exchange, J. Geophys. Res., 81, 2701-2708, 1976.
- Sonnerup, Bengt U.O.
- Magnetic field reconnection, p. 45-108 in vol. 3 of Solar System Plasma Physics, C.F. Kennel, L.J. Lanzerotti and E.N. Parker, eds., North Holland 1979.
- Sonnerup, Bengt U.O. et al.
- Evidence for magnetic field reconnection at the Earth's magnetopause, J. Geophys. Res., 86, 10,049-10,067, 1981.
- Spiro, Robert W. and Richard A. Wolf
- Electrodynamics of convection in the inner magnetosphere, in Magnetospheric Currents, T.A. Potemra, editor, p. 247-259, Geophysical Monograph 28, Amer. Geophys. Union, Washington, D.C., 1984.
- Spitzer, Lyman Jr.
- Physics of Fully Ionized Gases, Interscience, NY, 1956 (2nd ed. 1962).
- Stern, David P.
- The motion of magnetic field lines, Space Sci. Rev., 6, 147-173, 1966.
- Stern, David P.
- Large-scale electric fields in the Earth's magnetosphere, Rev. Geophys., 15, 156-194, 1977.
- Stern, David P.
- Energetics of the magnetosphere, Space Sci. Rev., 39, 193-213, 1984.
- Stern, David P.
- A conversation with Jim Dungey, Eos, 67, 1394-5, 1986.
- Stern, David P.
- A brief history of magnetospheric physics before the spaceflight era, Rev. Geophys., 27, 103-114, 1989.
- Stern, David P.
- The Substorm, Eos, 70, 130, 28 February 1989.
- Stern, David P.
- Substorm Electrodynamics, J. Geophys. Res., 95, 12,057-12067, 1990.
- Stern, David P.
- The beginning of substorm research, p. 11-14 in Magnetospheric Substorms, J.R.Kan, T.A.Potemra, S. Kokubun and T. Iijima, eds., Geophysical Monograph 64, Amer. Geophys. Union, Washington, DC 1991.
- Stern, David P.
- The linkage between the ionosphere and the plasma sheet, J. Geomag. Geoelectr., 44, 1109-1120, 1992.
- Stern, David P. and Mauricio Peredo
- The exploration of the magnetosphere, internet document http://www~spof.gsfc.nasa.gov/Education/Intro.html (world-wide web), 1995.
- Stone, Robert G.
- Research results from the Radio Astronomy Explorer, Proceedings of the AIAA 6th Annual Meeting (Anaheim, California 20-24 October, 1969; AIAA Paper 69-1049, 1969.
- Sugiura, Masahisa
- Identification of the polar cap boundary and the auroral belt in the high-latitude magnetosphere: A model for field-aligned currents, J. Geophys. Res., 80, 2057-2068, 1975.
- Sugiura, Masahisa and T.N. Davis
- Auroral electrojet activity index AE and its universal time variations, J. Geophys. Res., 71, 785-801, 1966.
- Svalgaard, Leif
- Sector structure of the interplanetary magnetic field and daily variation of the geomagnetic field at high latitudes, Geophys. Paper R-6, Danish Meteorolog. Inst., Copenhagen, 1968.
- Svalgaard, Leif
- Interplanetary magnetic-sector structure, 1926-1971, J. Geophys. Res., 77, 4027-4034, 1972.
- Svalgaard, Leif
- Polar cap magnetic variations and their relationship with the interplanetary magnetic sector structure, J. Geophys. Res., 78, 2064-2078, 1973.
- Sweet, Peter A.
- The effect of turbulence on a magnetic field, Mon. Not. Roy. Astr. Soc., 110, 69-83 , 1950.
- Sweet, Peter A.
- The neutral point theory of solar flares, p. 123-134 in Electromagnetic Phenomena in Cosmical Physics, B. Lehnert, ed., Cambridge U. Press, London 1958.
- Tsuruda, Koichiro, and Hiroshi Oya (c)
- Introduction to the Exos-D (Akebono) project, Geophys. Res. Lett., 18, 293-5, 1991.
- Tsyganenko, Nikolai A., D.P. Stern and Z. Kaymaz
- Birkeland currents in the plasma sheet, J. Geophys. Res., 98, 19,455-19,464, 1993.
- Van Allen, James A.
- Observation of high intensity radiation by satellites 1958 alpha and 1958 gamma, IOWA Univ. preprint SUI 60-13, reprinted in p. 58-75, Space Science Comes of Age, P.A. Hanle and V.D. Chamberlain, editors, Smithsonian Inst. Press, Washington, DC 1981.
- Van Allen, James A.
- Origins of Magnetospheric Physics, 144 pp., Smithsonian Inst. Press, Washington, DC 1983a.
- Van Allen, James A.
- Genesis of the International Geophysical Year, Eos, 64, 977, 1983b (reprinted on p. 49 in History of Geophysics, vol.1, C.S. Gillmor, ed., Amer. Geophys. Union, 1984).
- Van Allen, James, A.
- What is a space Scientist? An autobiographical example, Ann. Rev. Earth. Planet. Sci., 18, 1-26, 1990.
- Van Allen, James A. (ed.)
- Cosmic Rays, The Sun and Geomagnetism: The Works of Scott E. Forbush, 472 pp., AGU, Washington, DC, 1993.
- Van Allen, James A.
- Early rocket observations of auroral bremstrahlung and its absorption in the mesosphere, J. Geophys. Res., 100, 14,485-497, 1995.
- Van Allen, James A., George H. Ludwig, Ernest C. Ray and Carl E. McIlwain
- Observation of high intensity radiation by satellites 1958 Alpha and Gamma, Jet Propulsion, 28, 588-592, 1958.
- Van Allen, James A., C.E. McIlwain and G.H. Ludwig
- Radiation observations with satellite 1958 epsilon, J. Geophys. Res., 64, 271-286, 1959.
- Van Allen, James A. and L. A. Frank
- Radiation measurement to 658,300 km with Pioneer IV, Nature, 184, 219-224, 1959.
- Vasyliunas, V.M.
- A survey of low-energy electrons in the evening sector of the magnetosphere with OGO 1 and OGO 3, J. Geophys. Res., 73, 2839--2884, 1968.
- Vasyliunas, Vytenis M.
- Mathematical models of magnetospheric convection and its coupling to the ionosphere, p. 60-71 in Particles and Fields in the Magnetosphere, B.M. McCormac, ed., D. Reidel 1970.
- Vasyliunas, Vytenis M.
- The interrelationship of magnetospheric processes, p. 29-38 in Earth's Magnetospheric Processes, B.M. McCormac, ed., D. Reidel, 1972.
- Vasyliunas, Vytenis M.
- Theoretical Models of Magnetic Field Merging, 1, Rev. Geophys., 13, 303-336, 1975.
- Vasyliunas, Vytenis M. and Richard A. Wolf
- Magnetospheric substorms: Some problems and controversies, Rev. Geophys., 11, 181-189, 1973.
- Vernov, S.N., N.L. Grigorov, I.P. Ivanenko, A.I. Lebedinskii, V.S. Murzin and A.E. Chudakov
- Possible mechanism of production of "terrestrial corpuscular radiation" under the action of cosmic rays, Soviet Physics (Doklady), 4, 154-157, 1959 (in Russian Doklady 125, 304-307, 1959).
- Vestine, Ernest H. and S. Chapman
- The electric current system of geomagnetic disturbance, J. Geophys. Res., 43, 351-382, 1938.
- Viking Science Team
- The Viking program, Eos, 67, 793-795, 21 October 1986.
- Voigt, G.-Hannes, and R.-A. Wolf
- Quasi-static magnetospheric MHD processes and the "Ground State" of the magnetosphere, Rev. Geophys., 26, 823-843, 1988.
- Von Braun, Wernher
- The Redstone, Jupiter and Juno, in The History of Rocket Technology, pp. 107-121, E.M.Emme, editor, Wayne State University, Detroit 1964.
- Walen, C.
- On the distribution of the solar general magnetic field and remarks concerning the geomagnetism and the solar rotation, Arkiv fšr Matematik Astronomi och Fysik, 33-A, no. 18, 1946.
- Walker, Raymond J., T. Ogino, J. Raeder and M. Ashour-Abdalla
- A global magnetohydrodynamic simulation of the magnetosphere when the interplanetary magnetic field is southward: the onset of magnetotail reconnection, J. Geophys. Res., 98, 17,235-17,249, 1993.
- White, R. Stephen
- The Earth's radiation belts, Physics Today, 19, 25-38, October 1966.
- White, R. Stephen
- High energy proton radiation belt, Rev. Geophys., 11, 595-632, 1973.
- Wilcox, John M. and N.F. Ness
- A quasi-stationary co-rotating structure in the interplanetary medium, J. Geophys. Res., 70, 5793-5806, 1965.
- Wilcox, John M.
- The interplanetary magnetic field, Space Sci. Rev., 8, 258-328, 1968.
- Wilcox, John M.
- Inferring the interplanetary magnetic field by observing the polar geomagnetic field, Rev. Geophys., 10, 1003-1014, 1972.
- Williams, Donald J.
- Ring Current and Radiation Belts, Rev. Geophys. , 25, 570-578, 1987.
- Williams, Donald J., E.C. Roelof and D.G. Mitchell
- Global magnetospheric imaging, Rev. Geophys., 30, 183-208, 1992.
- Winckler, John R. and Laurence E. Peterson
- Large auroral effect on cosmic ray detectors observed at 8 g/cm2 atmospheric depth, Phys. Rev., 108, 903-4, 1957.
- Winckler, John R., Laurence E. Peterson, Roger Arnoldy and Robert Hoffman
- X rays from visible aurorae at Minneapolis, Phys. Rev., 110, 1221-1231, 1958.
- Wygant, John R., Roy B. Torbert and Forrest S. Mozer
- Comparison of S3-3 polar cap potential drops with the interplanetary magnetic field and models of magnetopause reconnection, J. Geophys. Res., 88, 5727-35, 1983.
- Yaeger, D.M. and L.A. Frank
- Low energy electron intensities at large distances over the Earth's polar cap, J. Geophys. Res., 81, 3966-3976, 1976.
- Zmuda, Alfred J., J.H. Martin and F.T. Heuring
- Transverse magnetic disturbances at 1100 km in the auroral region, J. Geophys. Res., 71, 5033-5045, 1966.
- Zmuda, Alfred J. and James C. Armstrong
- The diurnal flow pattern of field-aligned currents, J. Geophys. Res., 79, 4611-4619, 1974.
Captions of Figures
1. Explorer 1
2. Counting rate of Explorer 3 during a pass through the radiation belt. The highest rate was encountered during the segment of zero counts.
3. Counting rates of Pioneers 3 and 4, during traversals of the outer radiation belt [Van Allen and Frank, 1959].
4. Regions and currents of the Earth's magnetosphere, representing the state of our knowledge around 1970 [Lopez and Baker, 1994]. A small normal field component on the magnetopause allows some interplanetary field lines to link up with the Earth's field.
5a. Schematic view of the convection pattern produced in a closed magnetosphere by viscous-like forces, as envisioned by Axford and Hines.
5b. Schematic view of the convection in Figure 5a when mapped along field lines to the polar cap. Ideally, the contours are also equipotentials of the electric field, which near the center of the pattern is directed from dawn to dusk.
6. Magnetic merging at an X-type neutral line. Solid lines are magnetic field lines, dashed lines flow lines of the plasma (after Sonnerup [1979]).
7. Schematic view of Dungey's original view of the open magnetosphere, for a purely southward interplanetary magnetic field (IMF).
8. Transmission of electric fields along open field lines and to the cross-tail circuit.
9. Cartoon of the connection of the IMF to the magnetosphere, for various IMF orientations: (a) purely southward IMF; (b) southward slanting IMF (c) northward slanting IMF.
10. An example of the auroral energy spectrum, from Franck and Ackerson, [1971].
11. The proposed circuit of Birkeland currents, according to Schield et al. [1969]: JE are region 1 currents connected to the interplanetary field, JP are currents in the polar ionosphere, JB are region 2 currents and JA are currents of the partial ring current that close the circuit across midnight (the terms "region 1" and "region 2" were only introduced in 1976).
12. A straight current impinging on a uniformly conducting infinite flat sheet creates zero magnetic field underneath. By superposing two such patterns, with opposing straight currents, the property is extended to a current flowing into the (flat) ionosphere and out again.
13. A map of the polar ionosphere, showing the average configuration of Birkeland (field aligned) currents there. Regions where the current enters the ionosphere have dark shading, regions where it flows away from Earth and into space have light shading. From Iijima and Potemra, [1976b]; the origin is at the magnetic pole and the Sun's direction is on top.
14. Plot by Akasofu and Chapman [1963] of the magnetic disturbance associated with a magnetic storm, as observed near the equator (Honolulu) and in the auroral zone (College, in Fairbanks Alaska). The latter is punctuated by many "substorms."
15. The stretching of the Earth's magnetic tail during the "growth phase" which precedes a substorm, from Fairfield and Ness [1970].
16. Schematic view of the formation of a near-Earth neutral line (NENL) and a plasmoid, as proposed by the NENL scenario of substorms.
|