Nuclear Fission
Uranium nuclei in nature are unstable. Each of their 92 protons repels the rest, and sooner or later (half of them within 4.5 billion years) they eject a positive fragment, an "alpha particle" which is another term for a rapidly moving nucleus of helium. Almost all the helium atoms we extract from natural gas and from rocks--for filling balloons and for other uses--were originally created as alpha particles.
But there exists a way of speeding up this break-up, by exposing the material to free neutrons.
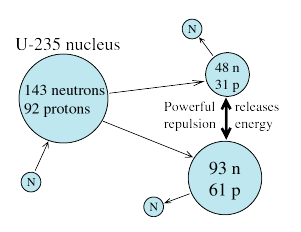
Free neutrons are not found in nature (they would decay into protons and electrons), but they can be released from atoms of beryllium by bombarding such atoms with alpha particles from radioactive materials, or by other methods. Since they are only attracted by nuclei (while protons are repelled before they get close enough for the nuclear attraction to dominate), they can easily enter a nucleus and stick to it--a bit like small magnets clicking onto a chunk of steel.
Such an attraction releases energy. If the nucleus is heavy and unstable, like that of uranium, adding energy destabilizes it even more, to where it may break up immediately. The interesting thing about such a break-up is the spectacular way in which it sometimes happens, as was discovered by Hahn and Meitner in 1939. Instead of breaking off a little chip, a helium nucleus containing less than 2% of the mass, the entire nucleus splits into 2 comparable fragments, typically containing 1/3 and 2/3 of the mass.
This process is called nuclear fission and besides the speed with which it can take place, it has at least two other remarkable features:
- It releases much more energy then the chipping-off of a helium nucleus ("alpha radioactivity").
- The fragments themselves are unstable. If you break up a nucleus which has (say) 40 protons for every 60 neutrons, into fragments whose optimal ratio is only (say) 45 to 55, there is bound to be some adjustment.
Ordinarily, such an adjustment calls for the conversion of some neutrons into protons (plus emitted electrons, "beta rays"), a process known as "beta radioactivity." Such an adjustment does in fact occur, making such fragments fiercely radioactive, and turning their disposal into a major issue for the nuclear power industry.
But at first, when the nucleus breaks up, the fragments are too unstable for such a gradual process. A quicker adjustment is needed, and the fragments achieve it by each emitting one or sometimes more free neutrons.
The Chain Reaction
On the average, about two neutrons are released this way per fission event. But it takes only one neutron to initiate another fission! Thus if fissionable nuclei are so densely packed that each neutron is bound to produce a new fission, the number of fission events quickly multiplies: 2, 4, 8, 16, 32, 64, 128... Since the energy release is proportional to the rate of fission, it also grows--very quickly!
This chain reaction is what makes a nuclear bomb (or "atomic bomb") function. The material with nuclei prone for fission--usually plutonium, an artificial heavy elements with 94 protons--must be compressed tightly and at the appropriate moment, exposed to a blast of neutrons. A variety of tricks, all of them top secret, is used to make sure that at least an appreciable fraction of its atoms undergoes fission before the whole thing blows apart.
Commercial nuclear power is produced somewhat differently, in a more controlled fashion. The fuel is uranium 235 (U-235)--a variant ("isotope") with 92 protons but only 143 neutrons, not 146, an odd number which makes it less stable. Natural uranium consists mostly of U-238, and it can absorb a neutron without undergoing fission (it ultimately turns into plutonium). U-238 therefore will not support a chain reaction. However, 0.7% of uranium is U-235, which can fission as soon as it absorbs a neutron.
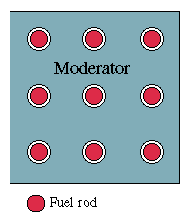
By using a clever design, one can actually build a reactor fueled by natural uranium. The trick is to form the fuel into rods, and put between them some material ("moderator") which slows down neutrons but does not absorb them, e.g. pure carbon or "heavy water" containing the heavy isotope of hydrogen. Neutrons produced in a rod generally escape into the moderator, and by the time they hit another rod, they are moving very slowly: such slow neutrons are gobbled up much more avidly by U-235 than by U-238, so that even in a rod containing only 0.7% U-235, the U-235 atoms make most of the "catches. "
The Critical Mass
It should be added that many neutrons are also lost--escaping from the edges of the reactor into the surrounding material, or being absorbed inside it by the " wrong " nuclei, the ones which do not undergo fission. In fact, a reactor needs to be carefully designed to sustain a chain reaction in the first place: but it can be done.
From the beginning, complex and very expensive methods were devised to separate U-235 or to enrich its percentage past 0.7%. Today many (though not all) commercial power reactors use enriched fuel, which makes the design of reactors easier and more controllable. With enriched fuel, ordinary water can serve as moderator, and it is even feasible to combine moderator and fuel, dissolving some uranium compound in water which acts both as moderator and as a distributor of heat. Canada's "CANDU" power reactors use ordinary uranium, but because ordinary water may absorb some neutrons, it is surrounded by "heavy" water (whose hydrogen is replaced by its heavy isotope, deuterium).
Such a reactor--or a chunk of plutonium--will not support a chain reaction if it is too small. If the amount of fissionable material is less than a critical mass, the average fission occurs too close to the surface. Even though (say) 2 neutrons are produced in each fission, on the average 1.2 of them escape to the outside before hitting another nucleus, leaving only 0.8 neutrons to continue the process, whereas one or more are needed.
When processing nuclear fuel, or reprocessing fuel rods, it is therefore essential to work only with small quantities to prevent any accidental chain reaction. On 30 September 1999, at the nuclear processing plant in Tokaimura, Japan, workers thought they would save time by combining several batches of a solution of uranium. With a flash of blue light, a chain reaction began, giving three workers very bad doses of radiation and lasting 18 hours. After 3 months one worker died (in spite of extreme measures), one was discharged from the hospital and one is still (as of 12/99) in intensive care.
A detailed report on the accident ("What Happened in Tokaimura?") appeared in "Physics Today", December 1999, p. 52-4. A similar accident occured in the US in the 1950s, when a worker extracting plutonium from a solution in one liquid into another took a shortcut and combined several batches. He died of radiation exposure within two days.
[A note about history: The first nuclear reactor was designed by Enrico Fermi and was built under the stands of an old stadium at the University of Chicago. Rather than rods, it used cylindrical pellets of uranium, and these were embedded in a big "pile" of bricks of pure carbon, the moderator. It achieved a self-sustaining chain reaction on 2 December, 1942, and the name "atomic pile" for a nuclear reactor remained in use for about a decade afterwards. ]
The Controlled Nuclear Reactor
Because a nuclear reactor requires neutrons that have slowed down, it has a built-in delay and cannot explode like a nuclear bomb (even if scary films claim otherwise). Still, the chain reaction can grow very rapidly, and unless it is controlled, the reactor could in principle heat up to where it melts down. The usual method of control is to insert among the fuel "control rods" which strongly aborb neutrons--e.g. of the metal cadmium, also used in electroplating. By absorbing free neutrons, these rods slow down or stop the chain reaction.
Fortunately, nature has been helpful here. About 1% of the neutrons released in fission are not emitted promptly but are delayed, by a fraction of a second (you can think of this process as a kind of radioactivity with very short half-life) . Reactors are always operated to produce just barely enough neutrons to sustain a chain reaction. If for some reason the heat output starts to rise, the delayed neutrons slow down the rate of increase to where an automatic mechanism lowering or raising the control rods is fast enough to stop it.
Power reactors in the US use ordinary water as a moderator, inside a "pressure vessel" made of thick steel, with rod-like fuel elements and control rods fitted through openings in its lid. To start the chain reaction
- the control rods are withdrawn part of the way,
- the fast fission fragments heat the fuel elements,
- the fuel elements heat the water, steam is produced (usually, "clean" steam in pipes separated from the radioactive reactor water), the steam turns turbines,
and - generators attached to the turbines produce electricity.
That is the basic process --the details are many and much more complicated.
Is this the energy of the future? As of this writing (1999) France gets 75% of its electric energy from nuclear power, and many industrial countries, short on coal and oil, also obtain an appreciable fraction of their energy this way--e.g. 1/3 of the energy used in Japan and Spain. In the US, after an enthusiastic start, the use of nuclear power has leveled off to about 20% of the power generated, mainly due to public resistance to nuclear energy.
The US however is fortunate in having large reserves of coal: its growing energy consumption is largely met by these fuels. Environmentally, the choice is between two alternatives:
- Burning coal and natural gas, which produces carbon dioxide (CO2) and other pollutants, and which may therefore amplifies the "greenhouse effect" and accelerate global warming; or else
- Using nuclear power stations, with the associated production of nuclear waste.
It is not easy to choose, and if we reject both options, we can expect much higher power costs and much less available power.
Updating in 2016 this web-page of 1999, much has already changed. Because CO2 promotes global heating, a strong movement has arisen to reduce the burning of coal; as intermediate measure, coal is being replaced by the methane of natural gas, which still contains carbon but also has hydrogen. Beyond this, photovoltaic power, generating electricity from sunlight, is rapidly rising, and may eclipse nuclear energy.
|
Nuclear Accidents
Suppose something went wrong with the cooling mechanism. Automatically, of course, control rods are lowered and any chain reaction stops immediately. But the radioactive waste in the reactor core continues to generate heat, so that cooling must still be provided for some hours, if not days. On 28 March 1979, at the nuclear power station at Three Mile Island, outside Harrisburg, Pennsylvania, a minor malfunction led to a series of errors, shutting down for a while both the main and emergency cooling systems.
The residual heat of the nuclear wastes melted part of the core and created (by chemical reaction) free hydrogen, which further complicated the situation. The billion-dollar reactor was a total loss, but the worst damage was probably to the public's trust in the safety of nuclear power. Still, the reactor vessel was not breached, and the second line of defense, the heavy concrete "containment building" inside which it was housed, also remained intact.
The power reactor at Chernobyl, near Kiev, capital of the Ukraine, had a different design--like Fermi's original reactor, it used a pile of carbon (graphite) to slow down neutrons, with pipes inside it carrying the fuel rods, control rods and cooling water. It was a big reactor, and was not enclosed in a containment building.
On 25 April 1986, operators lost control of an unwise engineering experiment at low power. The power level surged, the reactor vessel burst, and the hot steam and graphite (as well as combustible zirconium metal used in the fuel rods) reacted with hot steam and with oxygen of the atmosphere to produce an intense fire, whose plume rose to high altitudes and spread radioactive debris over a wide area. Of the fire-fighters called to extinguish the fire, many later died from radiation. Towns and villages near Chernobyl had to be evacuated (they remain empty 30 years later, although wildlife flourishes) and agricultural produce over much of Europe was contaminated. The remains of the reactor were later encased in a thick cover of concrete, entombing the radioactive waste left inside it. That cover has been wearing out, and an arched shed 300 feet tall was build nearby, resting on rails on which it can be slid to enclose the remains of the reactor
Since these accidents, nuclear power generation in the US has proceeded without any major mishap. However, nuclear waste is still kept in temporary storage, as the national policy for its treatment and disposal continues to be debated. The other reactor at at Three Mile Island (and even the ones at Chernobyl) have been restarted to supply power again.
One major nuclear accident occurred since then, in Japan, involving a group of nuclear power stations in the Fukushima district in Japan. A series of stations was built on high ground overlooking the sea, but their cooling water was chilled by seawater, using pumps near the seashore. On 11 March 2011 a severe earthquake struck the area and a large tsunami wave arose, destroying the cooling pumps and causing meltdown in the reactors. The tsunami devastated much of the district, which 5 years later remains evacuated and is closed due to leak of radioactive waste. Many power stations in Japan were shut down after the accident, and some remain closed.
An overview of "Nuclear Power Damage in Japan in the Earthquake of 3/2011" from The Washington Post, 3-12-2011 is linked here.
As the example of France suggests, nuclear power can be the main energy source of an industrial nation, though it calls for a high level of professional competence and carefully designed safety features. At the same time, the accidents at Three Mile Island and Chernobyl are a reminder that this power source carries unique risks of its own.
Further Exploring
If you have the time and the motivation, an enormous number of sources can give you more detailed information about matters discussed here, both in print and on the web. There is, for instance, "The Virtual Nuclear Tourist", a very detailed overview of the nuclear power industry.
Twenty five years after the accident at Three Mile Island, J. Samuel Walker, a historian with the Nuclear Regulatory Commission, published "Three Mile Island: A Nuclear Crisis in Historical Perspective (315 pp, $24.94, U. Calif Press, Berkeley, 2004). Its review is found in "Science,"vol. 305, p. 181, 9 July 2004.
Another rich source of information (also covering nuclear weapons) is the book "Megawatts and Megatons: A Turning Point in the Nuclear Age?" by Richard L. Garwin and Georges Charpak, 431 pp, $30, Knopf, New York, 2001.
A shorter, 4 page summary of nuclear power (comparable to this web page) is "Nuclear Fission: Atoms Unleashed", the center feature section ("Inside Science #157") betw. p. 28-9 in New Scientist, 18 January 2003.
Questions from Users:
***